Fructose, also known as fruit sugar, is a six-carbon monosaccharide, so it is a hexose.
It was discovered by the French chemist Dubrunfaut A-P. in 1847, the same person who discovered mutarotation three years earlier, and later, maltose, and later maltose.[1] In 1857, the English chemist Miller W.A. named it fructose, from the Latin fructus, which means fruit, followed by the suffix –ose to designate it as a carbohydrate.[2]
In solution, it occurs in both open-chain and cyclic form. Four stereoisomers are possible in the cyclic configuration.[3]
It comes almost exclusively from the diet. It is present in fruit and vegetables, honey, sucrose, and industrial foods such as invert sugar and high-fructose corn syrup.[4] In animals, it can be synthesized from glucose or sorbitol through the polyol pathway.[5]
Excessive intake may contribute to the development of gout.[6]
In humans, it is metabolized mainly in the liver by a pathway called fructolysis, with production of triose phosphates which can be used for both anabolic and catabolic purposes.[7]
Two inborn errors of fructose metabolism are known: essential fructosuria and hereditary fructose intolerance.[7][8]
Contents
- Chemical properties
- Food sources
- Intestinal absorption
- Blood fructose levels
- Hepatic fructolysis
- Fructose and gout
- Endogenous synthesis
- Inborn errors of fructose metabolism
- References
Chemical properties
Like the other hexoses, it has a molecular formula C6H12O6 and a molecular weight 180.156 g/mol.[9]
It can exist as two enantiomers, which, according to the D-L system or Fischer-Rosanoff convention, are called D-fructose and L-fructose. In nature, the D-enantiomer prevails, so the term fructose will be used to indicate D-fructose.
Like other monosaccharides, in solution it occurs in both open-chain and cyclic form. In the open-chain form, which may be represented by a Fischer projection, the carbonyl group at position 2 is part of a ketone group; hence, the monosaccharide is a ketohexose, unlike, for example, galactose, glucose, mannose and ribose which are aldohexoses, as the carbonyl group is part of an aldehyde group.
The carbonyl group makes fructose, like other monosaccharides, and maltose and lactose among the disaccharides, a reducing sugar.[10]
It is extremely soluble in water, about 3750 g/L at 20 °C, and among the monosaccharides, it is the most water-soluble.
Its high solubility accounts for its hygroscopicity, that is, the tendency to absorb water from the surrounding atmosphere.
This property has made it difficult to achieve the crystalline form, which has only recently been achieved with cheap and efficient methods.
In the crystalline form it appears as white crystals.[11]
Anomers
Anomerism is a type of optical isomerism characteristic of monosaccharides.
The open-chain form of fructose is thermodynamically unstable and is present only in trace amounts because of the cyclization due to the nucleophilic attack by the oxygen atom of one of the hydroxyl groups on the carbonyl carbon. This leads to the formation of a cyclic hemiketal, while the previous carbonyl carbon, bearing four different ligands, becomes an asymmetric center, i.e. a center of chirality, and is called the hemiketal carbon.[12]
The nucleophilic attack can occur from the bottom site or the topside of the sp2 plane of the carbonyl carbon, and, depending on the hydroxyl oxygen that performs the nucleophilic attack, four different configurations are possible, which differ in the configuration of the hemiketal carbon. This phenomenon is called mutarotation, and leads to the formation of two pairs of epimers:
- two furanose configurations, β-D-fructofuranose, and α-D-fructofuranose, namely six-member rings consisting of one oxygen atom and five carbon atoms;
- two pyranose configurations, β-D-fructopyranose and α-D-fructopyranose, namely, five-member rings consisting of one oxygen atom and four carbon atoms.
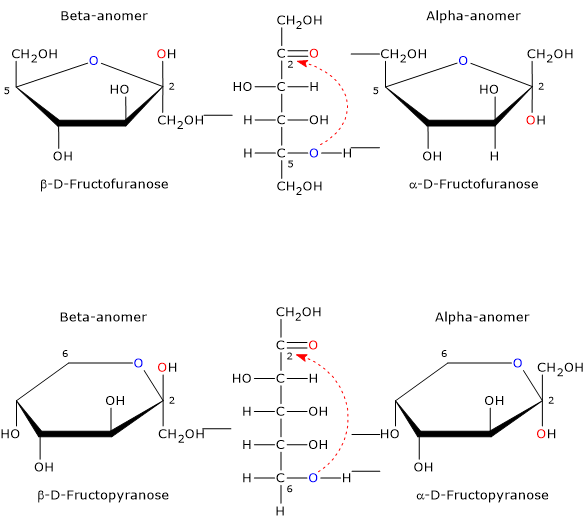
Epimers differing only in the configuration of the hemiketal carbon are called the alpha-anomer and the beta-anomer and the hemiketal carbon becomes the anomeric carbon or center.
Sweetness
It is the sweetest of the naturally occurring sugars, and is exceeded only by some food additives used as sweeteners.[13] However, its sweetness depends on its physical state, that is, whether it is in crystalline form or dissolved in water and, in the latter case, on water temperature. These differences are due to the predominant cyclic structure.
In crystalline form, only β-D-fructopyranose is present. This is the anomer responsible for the sweetness of the monosaccharide, and is twice as sweet as sucrose, which which consists of α-D-glucose, in pyranose form, and β-D-fructose, in furanose form.
When it is dissolved in solution, the sweetness varies according to the temperature of the solution. In fact, once dissolved, all four anomers are present, although α-D-fructopyranose is only found in trace amounts.[3]
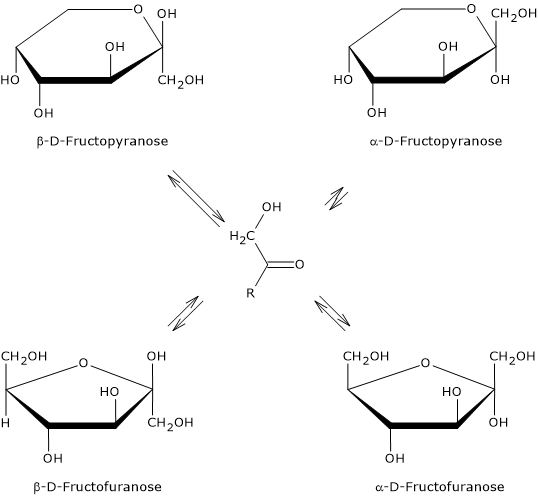
If β-D-fructopyranose prevails at 25 °C, representing about 68 percent of the anomers, as the temperature increases its percentage decreases and at 80 °C it drops to about 50%. Therefore, since furanose structures lack sweetness, as temperature increases its sweetening power decreases, and, for example, at 40 °C it has about the same sweetening power as sucrose, whereas at 60 °C it is about 20% less sweet.
Food sources
It is a natural component of the human diet, being present in fruit, where it may make up to 5 to 8% of the weight, and in lesser amounts in vegetables, especially tuberous vegetables such as potatoes and onions.[4]
Among fruits, grapes, apples, and pears contain good amounts, while the highest amount is found in dried figs, as a result of dehydration, and in apple and pear juices.
For thousands of years, before modernization and specialization in food processing and agricultural methods, humans had a fruit sugar intake estimated at 16–24 grams per day, mostly from fruit and honey.[13]
Currently, however, its per capita intake is estimated to range from 8 to 100 grams, with an average of about 80 grams in the USA. This increase is mainly derived from the consumption of the monosaccharide from food sources other than fruits and vegetables, such as:
- honey, where it makes up to 40% of the weight of the product;
- concentrated juices, for example, some homogenized fruits, where it makes over 60% of the weight of the product;
- invert sugar, an equimolar mixture of fructose and glucose obtained from the hydrolysis of sucrose;
- sucrose;
- molasses, where it makes up to 10% of the weight of the product;
- maple syrup, which contains about 63% by weight of sucrose;
- high fructose corn syrup (HFCS), where it makes up to 90% of the weight of the product. HFCS is used by the food industry, for example, to sweeten soft drink.[4]
Except for sucrose and sucrose-containing foods and beverages, in all other cases it is present in its free form. Notably, the richest source is the pure crystalline form, often used as a sweetener.
Intestinal absorption
In mammals, carbohydrate digestion occurs by means of hydrolases, such as the disaccharidases located in the brush border of enterocytes and pancreatic alpha-amylase. These enzymes hydrolyze the glycosidic bonds of disaccharides, oligosaccharides, and polysaccharides, releasing their constituent monosaccharides.[14]
The free fructose in the small intestine can arise from the hydrolysis of sucrose or be ingested in its free form.
The absorption of monosaccharides occurs in the small intestine, across the apical membrane of the enterocytes, and is mediated by specific transmembrane proteins that act as transporters. Fructose transporters belong to the glucose transporter family (GLUT family), proteins that mediate the facilitated diffusion of monosaccharides across the plasma membrane. In mammals, the GLUT family consists of 14 members, divided into three distinct classes, based on sequence homology and substrate selectivity.[15] Seven GLUT transporters are capable of transporting fructose, and in the intestine the most important are GLUT2 and GLUT5. The other five transporters, namely, GLUT7, GLUT9a/b, GLUT8, GLUT11, and GLUT12, have different degrees of fructose selectivity.[13]
Conversely, intestinal absorption of glucose and galactose is mainly mediated by the sodium-glucose linked transporter type I (SGLT1), a transmembrane protein belonging to the sodium-glucose cotransporter family. SGLT1 mediates active transport of the two monosaccharides across the apical membrane, using the transmembrane electrochemical gradient of sodium ions as an energy source.[16]
GLUT5
Fructose absorption occurs in the distal part of the duodenum and in the jejunum, and is mainly mediated by GLUT5, an insulin-independent transporter.[17]
Among the seven members of the GLUT family that can transport this monosaccharide, GLUT5 is the only one specific for it, showing a high affinity (Km ~6 mM), while it cannot transport glucose or galactose.[18] It is mainly found in the small intestine, like GLUT2, but also in the kidney, adipose tissue, brain, sperm, and skeletal muscle.[19]
In the prenatal phase, both mRNA levels of GLUT5 in enterocytes and the rate of fructose transport are very low, but they rapidly increase with weaning, regardless of diet. After weaning, mRNA levels of GLUT5 can be further induced by diets containing fructose.[20]
It seems that a diet rich in fruit sugar induces the intestinal synthesis of thioredoxin-interacting protein (TXNIP), a protein that binds and regulates GLUT5.[21]
ChREBP, a transcription factor that responds to carbohydrate intake, particularly glucose 6-phosphate levels, a key metabolite of glucose metabolism, has been shown to regulate TXNIP and intestinal GLUT5 expression, and is required for systemic tolerance to the monosaccharide.[22]
In the intestine, fructose is absorbed at a slower rate than glucose.[15] This could be partly due to the low activity of GLUT5 and GLUT2 for fructose, and to differences in the absorption process, as it is absorbed via passive transport.[19]
The intestinal absorption rate of fructose increases with chronic intake, due to the rapid up-regulation of the GLUT5 gene.[20]
GLUT2
Fructose release from the enterocytes, which occurs across the basolateral membrane, is mediated by GLUT2, the second contributor to its intestinal transport, also responsible for the release of glucose and galactose.[13]
It is a high capacity, low avidity transporter for glucose, fructose, and galactose, with Km values of approximately 20 mM, 67 mM and 96 mM, respectively, and, like GLUT5, is an insulin-independent transporter.[19]
Transporters | Approximate Km value | ||
---|---|---|---|
D-Glucose | D-Galactose | D-Fructose | |
SGLT1 | 0.5 | 1 | No interaction |
GLUT2 | 20 | 96 | 67 |
GLUT5 | Not determined | No interaction | 6 |
In addition to the intestine, GLUT2 is also found in the epithelial cells of the kidney, in the hepatocytes, where GLUT5 is poorly expressed, and in the beta cells of the pancreas.[2] In hepatocytes and renal epithelial cells, GLUT2 mediates fructose uptake and acts as a bidirectional facilitative transporter.
Moreover, in beta cells and hepatocytes, it also acts to sense blood glucose levels.[23]
Note: GLUT8 may contribute to the hepatic uptake of fructose.[24]
Glucose and intestinal absorption of fructose
The ability of the intestine to absorb fructose is saturable and ranges, in a healthy adult, from about 5 grams to more than 50 grams. Furthermore, it is influenced by the presence of glucose in the intestinal lumen.[25]
When fruit sugar is ingested alone, it is not absorbed very efficiently and, under physiological conditions, malabsorption symptoms appear in a dose-dependent manner, starting from a load of about 12 grams. However, the intake of fructose alone is quite rare, as it is normally ingested, for example, with sucrose or, during meals, with starch, a polysaccharide made up by glucose units.
Experimentally, it has been observed that the co-ingestion of glucose with 50 grams of fructose attenuates malabsorption symptoms in a dose-dependent manner, and that, with equimolar mixtures of the two monosaccharides, no malabsorption has been observed up to 100 grams of total sugars. Therefore, absorption is maximal when fructose is present in the lumen of the small intestine in an equimolar ratio with glucose. It is noteworthy that the biological mechanism underlying this phenomenon has not yet been clarified.[26]
These observations are very important, for example, in sports nutrition, as during competition or training, athletes may require a high intake of carbohydrates per unit of time, which may be achieved by mixtures of maltodextrin, i.e. glucose, and fructose.[27]
Gastrointestinal side-effects of unabsorbed fructose
Unabsorbed fructose increases the intraluminal osmotic pressure in the distal part of the small intestine and in the colon, drawing fluid into the lumen.[28]
Furthermore, like fiber, resistant starch and significant amounts of undigested or unabsorbed sugars, once in the colon, it can be fermented anaerobically by gut microbiota, which is part of the larger human microbiota.
All this leads to an excessive production of gases, such as methane, carbon dioxide, and hydrogen, and short-chain fatty acids, namely, fatty acids whose carbon chain length ranges from two to six atoms: acetic, propionic, butyric, and caproic acids.
In the intestine, the major short-chain fatty acids produced are acetic, propionic, butyric acids, in an approximate molar ratio of 60:20:20.[29] Many of these bacterial metabolites can affect intestinal motility and cause various gastrointestinal symptoms, such as bloating, stomach ache, diarrhea, and a general feeling of malaise. Of course, the net flow of water into the intestinal lumen, due to the presence of unabsorbed fructose, also contributes to these effects.[30]
Blood fructose levels
Once absorbed, fruit sugar enters the portal circulation and is mainly transported to the liver. Its entry into the circulation is slower than that of glucose, its levels are lower, but it persists longer in the bloodstream.[19]
In healthy adults, its serum concentration is approximately 0.008 mM, while its plasma concentration ranges from 0.03 to 0.04 mM.[13]
In healthy subjects who consume a high-fructose or high-sucrose diet:
- in peripheral circulation, plasma fructose levels can acutely increase up to tenfold, reaching values of 0.2–0.5 mM, therefore always in the micromolar range, and return to fasting levels within two hours;[31]
- in portal circulation, plasma concentration can reach the low millimolar range.[32]
Therefore, there is a steep concentration gradient of fructose between the blood entering and leaving the liver. This means that the liver, as well as the pancreas, is exposed to higher fructose concentrations than non-splanchnic organs such as the heart, skeletal muscle, and brain.[19]
Differences in blood levels of fructose and glucose
In the portal circulation, fructose concentration is much lower than that of glucose, which is approximately 5.5 mM. This difference arises from variations in intestinal absorption rate and hepatic clearance between the two monosaccharides.[33]
In the small intestine, fructose is absorbed at a slower rate than glucose.[19] This may be due, as previously seen, to the low activity of GLUT5 and GLUT2 towards fructose, and to the fact that its absorption into the plasma occurs via passive transport systems.
Hepatic clearance of fructose is significantly more efficient than that of glucose. The liver, which is the primary site of fructose metabolism, is able to:
- extract approximately 50–70% of an oral fructose load from the portal blood, whereas it extracts only 15–30% of an oral glucose load;
- return plasma fructose concentration to fasting levels within two hours after ingestion.
The kidneys also contribute to fructose clearance, albeit to a lesser extent than the liver, accounting for approximately 20%.[33]
Hepatic fructolysis
The major sites of fructose metabolism, or fructolysis, are the liver, and to a lesser extent, small intestine and kidneys.[7] This is due to the presence of specific enzymes required for its metabolism, namely, fructokinase (EC 2.7.1.4), fructose 1-phosphate aldolase or aldolase B (EC 4.1.2.13), and triose kinase or triokinase(EC 2.7.1.28). These enzymes catalyze the conversion of the monosaccharide into glycolytic and gluconeogenic intermediates, namely glyceraldehyde 3-phosphate and dihydroxyacetone phosphate.[10]
Once inside the hepatocyte, fructose is phosphorylated to fructose 1-phosphate by fructokinase, in a reaction that consumes one molecule of ATP.
Fructose + ATP → Fructose 1-phosphate + ADP + H+
Fructokinase has a low Km for fructose, around 0.5 mM, and can rapidly phosphorylate the monosaccharide. By contrast, fructose is a poor substrate for glucokinase (EC 2.7.1.2), which has an affinity for it about 20 times lower than for glucose. Furthermore, unlike hexokinase and phosphofructokinase-1, fructokinase activity is not regulated by the energy status of the cell.[18]
Unlike mammalian enzyme, plant fructokinase phosphorylates fructose at the 6-position to form fructose 6-phosphate, similar to the bacterial enzyme.[34]
Phosphorylation serves to trap the monosaccharide inside the cell, activate it for further metabolism, and, by lowering its cytosolic concentration, facilitate its continued uptake via facilitated diffusion.[19]
Fructose 1-phosphate is then cleaved to dihydroxyacetone phosphate and glyceraldehyde, in a reaction catalyzed by fructose 1-phosphate aldolase.
Fructose 1-phosphate → Dihydroxyacetone phosphate + Glyceraldehyde
While dihydroxyacetone phosphate is an intermediate of glycolysis and gluconeogenesis, glyceraldehyde must be phosphorylated to glyceraldehyde 3-phosphate to enter these metabolic pathway. This reaction is catalyzed by triose kinase and also requires ATP.[10]
Glyceraldehyde + ATP → Glyceraldehyde 3-phosphate + ADP + H+
The overall reaction is therefore:
Fructose + 2 ATP → 2 Glyceraldehyde 3-phosphate +2 ADP +2 H+
Regulation of hepatic fructolysis
Once triose phosphates are produced, the metabolic pathways of glucose and fructose converge. However, the steps leading to the conversion of fructose into two triose phosphate molecules do not involve glucokinase or phosphofructokinase-1. Consequently, these molecules enter the glycolytic pathway downstream of its primary regulatory checkpoints. This means that hepatic fructolysis is not modulated by the cellular energy charge nor by hormonal signals such as insulin and glucagon.[18]
Regulatory effects of fructose 1-phosphate
An increase in the intracellular concentration of fructose 1-phosphate affects hepatic glucose metabolism by modulating the activity of two key glycolytic enzymes: glucokinase and pyruvate kinase (EC 2.7.1.40).[35]
Fructose 1-phosphate enhances glucokinase activity by promoting its release from the glucokinase regulatory protein (GRP), which normally sequesters the enzyme in an inactive state within the nucleus. This facilitates glucose phosphorylation, cellular uptake, and promotes glycogen synthesis.[36]
Fructose 1-phosphate also acts as an allosteric activator of pyruvate kinase, contributing to the elevated blood lactate levels observed following fructose ingestion.[35]
In addition, fructose 1-phosphate can:
- enhance glycogen synthesis by allosterically inhibiting glycogen phosphorylase (EC 2.4.1.1), a key enzyme in glycogenolysis;[37]
- stimulate uric acid production by allosterically activating AMP deaminase (EC 3.5.4.6).[38]
Fate of triose phosphates
The triose phosphates derived from fructolysis can be directed toward gluconeogenesis, lipogenesis, or oxidative pathways.[39]
In the liver, the primary metabolic fate of fructose is conversion into glucose via gluconeogenesis. This newly synthesized glucose can either be released into the bloodstream or stored as glycogen, depending on current blood glucose levels.
- In fasting conditions, low concentrations of fructose-2,6-bisphosphate inhibit phosphofructokinase-1 (EC 2.7.1.11), thereby suppressing glycolysis, while activating fructose 1,6-bisphosphatase and promoting gluconeogenesis. Under these conditions, triose phosphates derived from fructose are predominantly used for gluconeogenesis, and the resulting glucose is released into the bloodstream to maintain normoglycemia.[18] A similar metabolic shift occurs during physical activity.
- Conversely, in the fed state, the triose phosphates are primarily directed toward glycogen synthesis.[19]
A portion of fructose may also undergo complete oxidation to carbon dioxide and water via the Krebs cycle, contributing to ATP production. Alternatively, it may be converted to lactate, which is then released into the circulation. This process, resembling a reverse Cori cycle, may be beneficial for working muscle tissue by providing an additional energy substrate.[40]
A minor portion of the triose phosphates may serve as precursors to fatty acid synthesis, and subsequently for triglyceride formation.[41]
Finally, it has been observed that acute fructose ingestion in healthy individuals is associated with a reduction in circulating free fatty acid levels. This may result from increased fatty acid clearance or inhibition of lipolysis; however, the exact mechanism remains unclear.[39]
Fructose and gout
Gout is an inflammatory condition of the joints caused by the deposition of uric acid crystals resulting from elevated circulating uric acid levels.
Gout has also been associated with high intakes of fructose.[40]
Why?
In the liver, the rapid phosphorylation of fructose may reduce intracellular free phosphate, which, in turn, promotes uric acid production through the activation of AMP deaminase, a process also stimulated by fructose 1-phosphate. The rapid phosphorylation of the monosaccharide lowers ATP levels and increases AMP levels. AMP deaminase, by converting AMP to IMP, directs the nucleotide toward conversion into uric acid.[37]
Finally, fructose may contribute to uric acid production by stimulating purine synthesis.[42]
Endogenous synthesis
Although fructose is mainly derived from diet, animals are able to produce it through the polyol pathway.[43] This pathway, present in various tissues and organs, converts glucose into fructose via sorbitol.[5]
- In the first step, glucose is reduced to sorbitol in a reaction catalyzed by aldose reductase (EC 1.1.1.21), a NADPH-dependent reductase with broad specificity for monosaccharides. In fact, it can also reduce galactose to galactitol.
- In the second step, sorbitol is oxidized to fructose in a reaction catalyzed by sorbitol dehydrogenase (EC 1.1.1.14), a NADH-dependent enzyme.
It is also possible to synthesize the monosaccharide directly from sorbitol, which is widely distributed in fruits and vegetables.
Endogenously synthesized fructose is the primary energy source for sperm and may play an important role in fertility.[44]
Inborn errors of fructose metabolism
Two inborn errors affect fructose metabolism: essential or benign fructosuria and hereditary fructose intolerance, both of which are autosomal recessive diseases.
- Essential or benign fructosuria is caused by a deficiency of hepatic fructokinase.
First described in 1876, it is an asymptomatic and benign condition that often goes undetected. Following the ingestion of fructose, sucrose, or sorbitol, elevated blood fructose levels are observed. The monosaccharide is partially excreted in the urine, while the remainder can be phosphorylated by hexokinase in muscle and adipose tissue to form fructose 6-phosphate.
Due to its benign nature, no dietary restrictions are necessary.[7] - Hereditary fructose intolerance usually manifests at the time of weaning, when foods containing the monosaccharide are introduced into the diet.[8]
It is caused by a deficiency of fructose 1-phosphate aldolase. Because of high fructokinase activity, a cytosolic accumulation of fructose 1-phosphate and phosphate trapping occur. This leads to a decrease in postprandial blood glucose levels due to the inhibition of both glycogenolysis and gluconeogenesis. Furthermore, the high consumption of ATP, used as the phosphate donor in the reaction catalyzed by fructokinase, and its limited regeneration result in ATP depletion. This, in turn, leads to an increased release of magnesium ions and elevated uric acid production.
This inborn error causes renal and liver dysfunction, and can be fatal. However, affected individuals develop symptoms only when exposed to fructose. Thus, the recomended therapy is a fructose-free diet.[7]
References
- ^ Dubrunfaut A. P. Sur une propriété analytique des fermentations alcoolique et lactique, et sur leur application à l’étude des sucres. Annales de Chimie et de Physique 1847;21:169-178
- ^ Miller W.A. Elements of chemistry: theoretical and practical. Part III. John W. Parker and Son. 1857
- ^ a b Shallenberger R.S. Intrinsic chemistry of fructose. Pure Appl Chem 1978;50(11-12):1409-1420. doi:10.1351/pac197850111409
- ^ a b c Ziesenitz S.C. Authorised EU health claim for fructose in foods, nutrients and food ingredients with authorised EU health claims: Volume 2, 2015 doi:10.1016/B978-1-78242-382-9.00011-6
- ^ a b Oates P.J. Polyol pathway and diabetic peripheral neuropathy. Int Rev Neurobiol 2002;50:325-92. doi:10.1016/s0074-7742(02)50082-9
- ^ Choi H.K., Curhan G. Soft drinks, fructose consumption, and the risk of gout in men: prospective cohort study. BMJ 2008;336(7639):309-12. doi:10.1136/bmj.39449.819271.BE
- ^ a b c d e Tran C. Inborn errors of fructose metabolism. What can we learn from them? Nutrients 2017;9(4):356. doi:10.3390/nu9040356
- ^ a b Kim M., Astapova I.I., Flier S.N., Hannou S.A., Doridot L., Sargsyan A., Kou H.H., Fowler A.J., Liang G., Herman M.A. Intestinal, but not hepatic, ChREBP is required for fructose tolerance. JCI Insight 2017;2(24):e96703. doi:10.1172/jci.insight.96703
- ^ National Center for Biotechnology Information. PubChem Compound Summary for CID 2723872, D-Fructose. https://pubchem.ncbi.nlm.nih.gov/compound/fructose. Accessed Dec. 18, 2023.
- ^ a b c Nelson D.L., Cox M.M. Lehninger. Principles of biochemistry. 6th Edition. W.H. Freeman and Company, 2012.
- ^ American Chemical Society. Fructose. 2017 https://www.acs.org/molecule-of-the-week/archive/f/fructose.html
- ^ Soderberg T. Organic chemistry with a biological emphasis. Volume II. Chemistry Publications. 2019.
- ^ a b c d e Douard V., Ferraris R.P. Regulation of the fructose transporter GLUT5 in health and disease. Am J Physiol Endocrinol Metab 2008;295(2):E227-37. doi:10.1152/ajpendo.90245.2008
- ^ Rosenthal M.D., Glew R.H. Medical biochemistry: human metabolism in health and disease. A John Wiley & sons, Inc., Publication, 2009.
- ^ Uldry M., Thorens B. The SLC2 family of facilitated hexose and polyol transporters. Pflugers Arch 2004;447(5):480-9. doi:10.1007/s00424-003-1085-0
- ^ Wright E.M., Hirayama B.A., Loo D.F. Active sugar transport in health and disease. J Intern Med 2007;261(1):32-43. doi:10.1111/j.1365-2796.2006.01746.x
- ^ Hajduch E., Litherland G.J., Turban S., Brot-Laroche E., Hundal H.S. Insulin regulates the expression of the GLUT5 transporter in L6 skeletal muscle cells. FEBS Lett. 2003;549(1-3):77-82. doi:10.1016/s0014-5793(03)00773-7
- ^ a b c d Hannou S.A., Haslam D.E., McKeown N.M., Herman M.A. Fructose metabolism and metabolic disease. J Clin Invest 2018;128(2):545-555. doi:10.1172/JCI96702
- ^ a b c d e f g Laughlin M.R. Normal roles for dietary fructose in carbohydrate metabolism. Nutrients 2014;6(8):3117-3129. doi:10.3390/nu6083117
- ^ a b Ferraris R.P. Dietary and developmental regulation of intestinal sugar transport. Biochem J. 2001;360(Pt 2):265-76. doi:10.1042/0264-6021:3600265
- ^ Dotimas J.R., Lee A.W., Schmider A.B., Carroll S.H., Shah A., Bilen J., Elliott K.R., Myers R.B., Soberman R.J., Yoshioka J., Lee R.T. Diabetes regulates fructose absorption through thioredoxin-interacting protein. Elife 2016;5:e18313. doi:10.7554/eLife.18313
- ^ Kim M., Moon J.S., Kim M.J., Seong M.W., Park S.S., Ko J.S. Hereditary fructose intolerance diagnosed in adulthood. Gut Liver. 2021;15(1):142-145. doi:10.5009/gnl20189
- ^ Cura A.J., Carruthers A. Role of monosaccharide transport proteins in carbohydrate assimilation, distribution, metabolism, and homeostasis. Compr Physiol 2012;2(2):863-914. doi:10.1002/cphy.c110024
- ^ DeBosch B.J., Chen Z., Saben J.L., Finck B.N., Moley K.H. Glucose transporter 8 (GLUT8) mediates fructose-induced de novo lipogenesis and macrosteatosis. J Biol Chem 2014;289(16):10989-10998. doi:10.1074/jbc.M113.527002
- ^ Latulippe M.E., Skoog S.M. Fructose malabsorption and intolerance: effects of fructose with and without simultaneous glucose ingestion. Crit Rev Food Sci Nutr 2011;51(7):583-92. doi:10.1080/10408398.2011.566646
- ^ Riby J.E., Fujisawa T., Kretchmer N. Fructose absorption. Am J Clin Nutr 1993;58(5 Suppl):748S-753S. doi:10.1093/ajcn/58.5.748S
- ^ Fuchs C.J., Gonzalez J.T., van Loon L.J.C. Fructose co-ingestion to increase carbohydrate availability in athletes. J Physiol 2019;597(14):3549-3560. doi:10.1113/JP277116
- ^ Holtug K., Clausen M.R., Hove H., Christiansen J., Mortensen P.B. The colon in carbohydrate malabsorption: short-chain fatty acids, pH, and osmotic diarrhoea. Scand J Gastroenterol 1992;27(7):545-52. doi:10.3109/00365529209000118
- ^ Cummings J.H., Pomare E.W., Branch W.J., Naylor C.P., Macfarlane G.T. Short chain fatty acids in human large intestine, portal, hepatic and venous blood. Gut 1987;28(10):1221-7. doi:10.1136/gut.28.10.1221
- ^ Skoog S.M., Bharucha A.E. Dietary fructose and gastrointestinal symptoms: a review. Am J Gastroenterol 2004;99(10):2046-50. doi:10.1111/j.1572-0241.2004.40266.x
- ^ Preston G.M., Calle R.A. Elevated serum sorbitol and not fructose in type 2 diabetic patients. Biomark Insights 2010;5:33-8. doi:10.4137/bmi.s4530
- ^ Sugimoto K., Hosotani T., Kawasaki T., Nakagawa K., Hayashi S., Nakano Y., Inui H., Yamanouchi T. Eucalyptus leaf extract suppresses the postprandial elevation of portal, cardiac and peripheral fructose concentrations after sucrose ingestion in rats. J Clin Biochem Nutr 2010;46(3):205-11. doi:10.3164/jcbn.09-93
- ^ a b Mayes P.A. Intermediary metabolism of fructose. Am J Clin Nutr 1993;58(5 Suppl):754S-765S. doi:10.1093/ajcn/58.5.754S
- ^ Stein O., Granot D. Plant fructokinases: evolutionary, developmental, and metabolic aspects in sink tissues. Front Plant Sci 2018;9:339. doi:10.3389/fpls.2018.00339
- ^ a b Eggleston L.V., Woods H.F. Activation of liver pyruvate kinase by fructose-1-phosphate. FEBS Lett 197015;6(1):43-45. doi:10.1016/0014-5793(70)80038-2
- ^ McGuinness O..P., Cherrington A.D. Effects of fructose on hepatic glucose metabolism. Curr Opin Clin Nutr Metab Care 2003;6(4):441-8. doi:10.1097/01.mco.0000078990.96795.cd
- ^ a b Van Den Berghe G., Hue L., Hers H.G. Effect of administration of the fructose on the glycogenolytic action of glucagon. An investigation of the pathogeny of hereditary fructose intolerance. Biochem J 1973;134(2):637-45. doi:10.1042/bj1340637
- ^ Lanaspa M.A., Tapia E., Soto V., Sautin Y., Sánchez-Lozada L.G. Uric acid and fructose: potential biological mechanisms. Semin Nephrol 2011;31(5):426-32. doi:10.1016/j.semnephrol.2011.08.006
- ^ a b Ter Horst K.W., Serlie M.J. Fructose consumption, lipogenesis, and non-alcoholic fatty liver disease. Nutrients 2017;9(9):981. doi:10.3390/nu9090981
- ^ a b Tappy L., Rosset R. Fructose metabolism from a functional perspective: implications for athletes. Sports Med 2017;47(Suppl 1):23-32. doi:1007/s40279-017-0692-4
- ^ Tappy L., Lê K.A. Metabolic effects of fructose and the worldwide increase in obesity. Physiol Rev 2010;90(1):23-46. doi:1152/physrev.00019.2009
- ^ Raivio K.O., Becker M.A., Meyer L.J., Greene M.L., Nuki G., Seegmiller J.E. Stimulation of human purine synthesis de novo by fructose infusion. Metabolism 1975;24(7):861-9. doi:10.1016/0026-0495(75)90133-x
- ^ Hwang J.J., Jiang L., Hamza M., Dai F., Belfort-DeAguiar R., Cline G., Rothman D.L., Mason G., Sherwin R.S. The human brain produces fructose from glucose. JCI Insight 2017;2(4):e90508. doi:10.1172/jci.insight.90508
- ^ Frenette G., Thabet M., Sullivan R. Polyol pathway in human epididymis and semen. J Androl 2006;27(2):233-9. doi:10.2164/jandrol.05108