Glycogen metabolism consists of two phases: a constructive phase in which glycogen synthesis occurs, and a degradative phase, known as glycogenolysis, in which the polysaccharide is catabolized.
Glycogenolysis releases glucose when it is needed.
In the liver, glycogen is a glucose reserve for the maintenance of normal blood glucose levels, and its breakdown occurs primarily:
- in the fasted state, e.g. during the nocturnal fast;
- between meals;
- during a high intensity physical activity.
In hepatocytes, glycogenolysis is stimulated by glucagon and adrenalin, inhibited by insulin, and subject to negative allosteric regulation by glucose as well.
Glycogen is an energy source for muscular activity; therefore, glycogen breakdown occurs during contraction, and only in muscles involved in the activity.
In muscle cells, glycogenolysis is stimulated by adrenaline, and regulated by positive and negative allosteric effectors, AMP and calcium ion, and ATP and glucose 6-phosphate, respectively.
Contents
- Steps of glycogenolysis
- Covalent regulation in muscle and liver
- Allosteric regulation oin muscle and liver
- References
Steps of glycogenolysis
Glycogenolysis begins by the action of glycogen phosphorylase (EC 2.4.1.1), a homodimer that for its activity requires the presence of pyridoxal-5-phosphate, a derivative of pyridoxine or vitamin B6. The enzyme catalyzes the phosphorolytic cleavage of α-(1,4) glycosidic bond, releasing glucose molecules one at a time from the non-reducing ends, that is, the ends with a free 4’-OH group, of the external branches. This reaction, which does not consume ATP but an orthophosphate, yields glucose 1-phosphate.
Glycogen(n glucose residues) + Pi → Glucose 1-Phosphate + Glycogen(n-1 glucose residues)
Note: in the small intestine, pancreatic alpha-amylase (EC 3.2.1.1) catalyzes the hydrolytic cleavage of the α-(1,4) glycosidic bonds of the starch, and yields glucose molecules.
In vivo, glycogen phosphorylase catalyzes an irreversible phosphorolysis, a particularly advantageous reaction for skeletal muscle and heart. The irreversibility of the reaction is ensured by the ratio [Pi]/[glucose 1-phosphate], which is usually greater than 100. Conversely, the reaction is easily reversible in vitro.
Glycogen phosphorylase acts repetitively on the non-reducing ends of branches, coming to a halt when the glucose unit that is 4 residues away from the branch point is reached: this is the outer limit of the limit dextrin. At this point, two enzymatic activities, present on the same polypeptide chain, complete glycogen breakdown: the α-(1,4)-glucan-6-glycosyltransferase (EC 2.4.1.25) and the amylo-α-(1,6)-glucosidase or debranching enzyme (EC 3.2.1.33). The first enzymatic activity transfers three of the remaining four glucose units from the branch to the non-reducing end of another branch, leaving in the first chain only a single glucose unit, that is attached to the chain by an α-(1,6)-glycosidic bond. The second enzymatic activity hydrolyzes this α-(1,6)-glycosidic bond, releasing glucose and an unbranched chain of α-(1,4)-linked glucose units.
Without the branch, glycogen phosphorylase can continue to remove glucose units until it reaches the next limit dextrin.
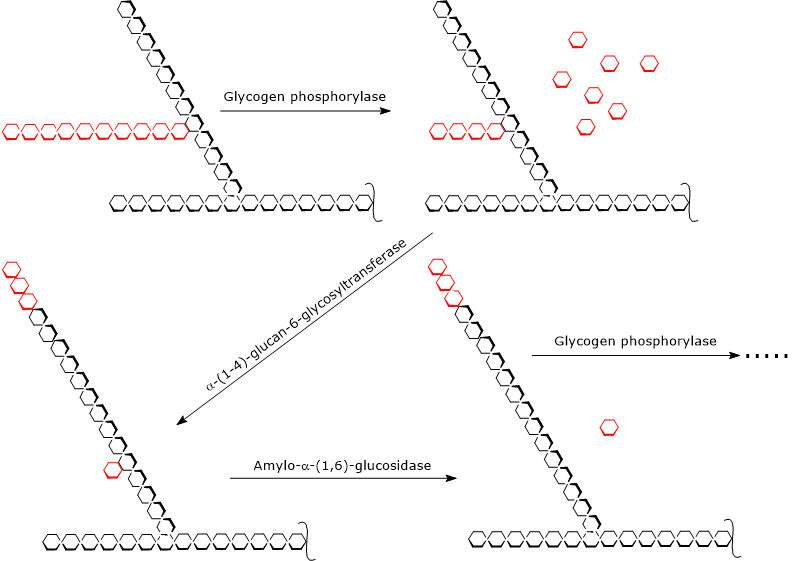
Therefore, the products of the reactions catalyzed by the three enzymatic activities are:
- glucose 1-phosphate, about 90 percent of the glucose molecules released);
- a small amount of free glucose, the remaining 10 percent, which are the 1,6 linked residues; in the muscle, hexokinase activity (EC 2.7.1.1) is so high that any free glucose molecule is phosphorylated to glucose 6-phosphate, and therefore activated, and metabolized within the cell;
- a smaller and less branched glycogen molecule.
Metabolic fate of glucose 1-phosphate in muscle and liver
Glucose 1-phosphate is a charged molecule, and therefore it is trapped within the cell.
It is converted to glucose 6-phosphate in the reaction catalyzed by phosphoglucomutase (EC 5.4.2.2), the same enzyme that also intervenes in glycogen synthesis converting glucose 6-phosphate to glucose 1-phosphate. This enzyme catalyses a reversible reaction: the direction is determined by the relative concentrations of the two molecules, and in this case moves the phosphate group from C1 to C6.
Glucose 1-Phosphate ⇄ Glucose 6-Phosphate
In the muscle, and in most of the other organs and tissues, glucose from glycogenolysis enters glycolysis as glucose 6-phosphate, bypassing the activation step catalyzed by hexokinase. Therefore, glycogen phosphorylase, releasing an already activated glucose molecule, saves an ATP. An ATP molecule is required to synthesize another glycolytic intermediate, the fructose 1,6-bisphosphate.
In this way, some of the activation energy required for glycogen synthesis is conserved: the net yield of ATP per glucose molecule by glycolysis to lactate is 3 rather than 2, an advantage for the working muscle. The overall equation is:
Glycogen(n glucose residues) + 3 ADP + 3 Pi → Glycogen(n-1 glucose residues) + 2 Lactate + 3 ATP
In the liver, glucose 6-phosphate from glycogen is dephosphorylated by glucose 6-phosphatase (EC 3.1.3.9), and then released into the bloodstream. These are the steps in the removal of glucose units, in the form of phosphorylated glucose, by hepatic glycogenolysis:
Glycogen(n glucose residues) + Pi → Glucose 1-Phosphate + Glycogen(n-1 glucose residues)
glucose-1-phosphate → glucose-6-phosphate
glucose-6-phosphate + H2O → glucose + Pi
The overall equation is:
Glycogen(n glucose residues) + H2O → Glycogen(n-1 glucose residues) +Gglucose
Covalent regulation in muscle and liver
Glycogen breakdown is under a fine control through covalent and/or allosteric modifications of some key proteins, such as phosphorylase kinase (EC 2.7.11.19), glycogen phosphorylase, and protein phosphatase 1.
Here, the effects of two hormones, which act through covalent modifications of target proteins, are analyzed:
- adrenaline, also known as epinephrine, produced by the adrenal glands, which acts for example on muscle, liver, and fat cells;
- glucagon, produced by alpha-cells of the pancreas, which acts on hepatocytes and adipocytes.
These hormones, binding to their membrane receptors, trigger an identical cascade of intracellular events that amplify by several orders of magnitude their signal, stimulating glycogenolysis and inhibiting glycogen synthesis.
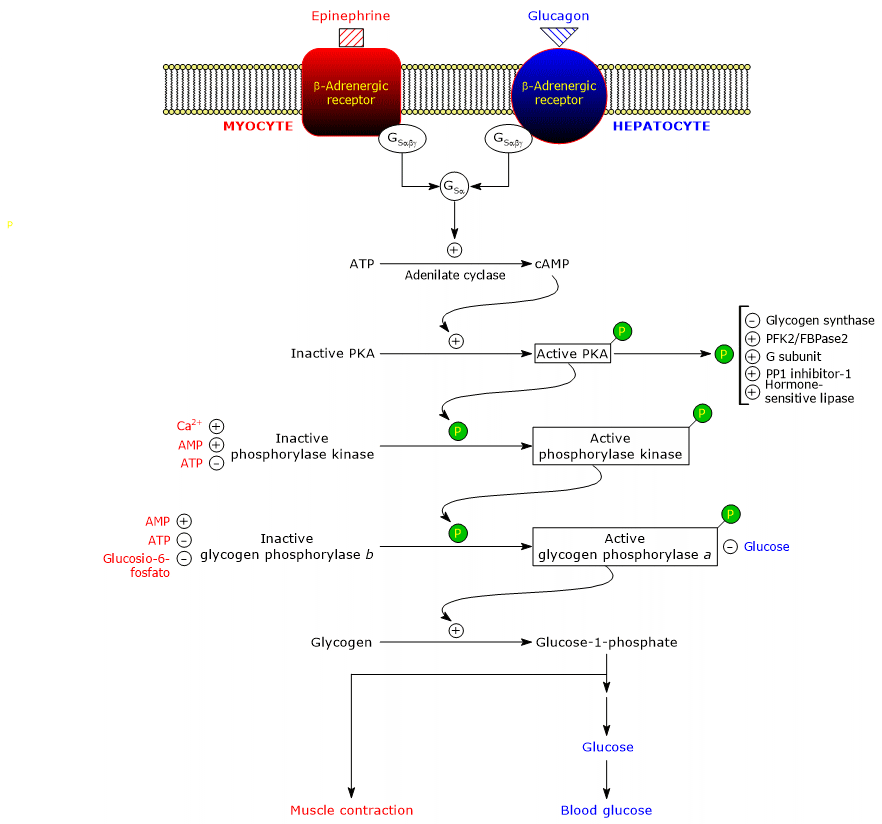
It should be noted that even acetylcholine, by the binding to the receptor located at the neuromuscular junction, triggers the same cascade of activations of adrenaline and glucagon.
Here, the proteins involved in the cascade.
Beta-adrenergic receptors
The receptors for adrenaline and glucagon are integral membrane proteins, with seven transmembrane α-helices.
The term “adrenergic” derives from adrenaline. There are four subtypes of adrenergic receptors: alpha1, alpha2, beta1 and beta2. In the subsequent discussion, only beta1 and beta2 receptors, referred to as beta, and which act in the same way, will be considered.
beta-adrenergic receptors cause changes in energy metabolism, such as:
- an increase in glycogen breakdown in muscle and liver cells;
- an increase in triglyceride breakdown, that is, lipolysis in adipose tissue.
Stimulatory G proteins
The binding of the hormone to the receptor causes a conformational change in the cytosolic portion of the receptor, and this modifies the interaction with the second protein in the cascade: the stimulatory guanine nucleotide binding protein or, more simply, stimulatory G protein (GS). It is a heterotrimer composed of three subunits: alpha, which contains the nucleotide binding site, beta, and gamma. In the inactive form, GSαβγ-GDP, the heterotrimer is coupled to beta-adrenergic receptors.
The conformational changes in the receptor allow it to catalyze the replacement of GDP with GTP in the alpha subunit of GSαβγ complex. This leads to the dissociation of the trimer into a inactive dimer, beta-gamma, and the GSα-GTP complex that moves along the plane of the inner surface of the plasma membrane, to which it is anchored by a covalently-linked palmitoyl group, until it reaches adenylyl cyclase (EC 4.6.1.1).
Note: the action of GS resembles those of the Ras proteins, another class of G-proteins which are involved in insulin signal transduction.
Adenyl cyclase
It is an integral membrane enzyme, whose active site is on the cytosolic side of the plasma membrane. The interaction between GSα and adenyl cyclase activates the enzyme that, in turn, catalyzes the synthesis of cAMP from ATP. This leads to an increase in the intracellular concentration of the cyclic nucleotide.
The stimulatory activity of GSα is self-limiting, as it is a GPTasi, that is, it hydrolyzes the bound GTP to GDP, thereby turning itself off. In the inactive form, GSα dissociates from adenyl cyclase and reassociates with the Gβγ dimer. Therefore, the heterotrimer is again available to interact with a hormone-receptor complex.
Protein kinase A
cAMP binds and activates cAMP-dependent protein kinase or protein kinase A or PKA (EC 2.7.11.11). The inactive form of the enzyme is a tetramer made up of two catalytic subunits and two regulatory subunits. Each of the two regulatory subunits has an autoinhibitory domain, that is, a region that occupies the binding site for the substrate of each catalytic subunit. The binding of two cAMP molecules to two sites on each regulatory subunit leads to a conformational change that causes their dissociation from tetramer, releasing the two catalytic subunits as active enzymes. The active form of PKA catalyzes the phosphorylation of some proteins, activating or inhibiting them, such as:
- glycogen synthase (EC 2.4.1.11), inhibited;
- hormone-sensitive lipase (EC 3.1.1.79), activated;
- phosphofructokinase 2/fructose-2,6-bisphosphatase (EC 2.7.1.105 and EC 3.1.3.46 respectively), activated;
- inhibitor-1 and the glycogen-binding (G)-subunit of protein phosphatase 1, activated;
- phosphorylase kinase, activated.
cAMP has a very short half-life: it is hydrolyzed to AMP, that has no second messenger activity, in the reaction catalyzed by cyclic nucleotide phosphodiesterase (EC 3.1.4.53). Caffeine and theophylline, two methylxanthines contained in coffee and tea, respectively, inhibit the phosphodiesterase, thereby increasing the half-life of cAMP, and enhancing its effects.
Phosphorylase kinase
The next step in the cascade is catalyzed by phosphorylase kinase.
This protein is made up of four different subunits, each present with four copies to form a complex referred to as (αβγδ)4. The γ subunits have catalytic activity, whereas alpha, beta and gamma are regulatory subunits. Alpha and beta subunits are phosphorylated when the enzyme switches from the inactive to the active state. The delta subunit, also called calmodulin, is a regulatory protein that binds calcium ions. This protein is also present in a large number of other enzymes as well. It acts as a calcium sensor, that is, it responds to changes in intracellular calcium concentration, influencing the activity of proteins with which it interacts. Phosphorylase kinase exists in two isoforms, one expressed in the liver and the other in skeletal and cardiac muscle; they differ with regard to the alpha and gamma subunits, which are encoded by different genes.
One of the target proteins of the phosphorylase kinase is glycogen phosphorylase. This enzyme exists as isoenzymes in different tissues, and in two conformational states in dynamic equilibrium, referred to as:
- T, for tense or taut, which is less active;
- R, for relaxed, which is more active, and able to bind to glycogen, also in the phosphorylated state.
Phosphorylase kinase phosphorylates a single serine residue (Ser-14) in each of the two subunits of glycogen phosphorylase, which is almost entirely in the T state, converting it to the active form, which, conversely, is almost entirely in the R state, and therefore triggering the breakdown of glycogen.
The phosphorylated enzyme is the more active form of the enzyme and is referred to as glycogen phosphorylase a; the non-phosphorylated enzyme is the less active form of the enzyme, and is referred to as glycogen phosphorylase b. The two enzymatic forms can be inhibited or activated allosterically.
In the muscle, glycogenolysis releases glucose 1-phosphate which, as previously seen, is metabolized in the muscle itself to produce energy for muscle contraction, and therefore for the fight-or-flight response triggered by adrenaline.
In the liver, glucagon triggers the release of glucose into the circulation to counteract hypoglycemia.
Once the stressful situation ends, phosphorylase a phosphatase, also called phosphoprotein phosphatase 1 or PP1 (EC 3.1.3.17), catalyzes the removal of phosphate groups from phosphorylase kinase and glycogen phosphorylase a, thus converting them to inactive forms. The enzyme removes the phosphate groups from glycogen synthase, as well. PP1 is made up of a catalytic subunit, which has low catalytic efficiency and low affinity for glycogen, and the aforementioned G-subunit, which belongs to a family of proteins that bind other proteins to glycogen, known as glycogen-targeting proteins (also phosphorylase kinase, glycogen phosphorylase, and glycogen synthase are bound to glycogen particles by proteins of this family).
PP1 is also inhibited by another protein called inhibitor 1 of PP1.
As previously seen, PKA phosphorylates:
- G-subunit, which, in the phosphorylated form, is not able to bind the catalytic subunit of PP1, and therefore PP1 does not meet its glycogen-associated targets (conversely, G-subunit phosphorylation induced by insulin stimulation, affecting diverse amino acid residues, allows the binding to the catalytic subunit of PP1);
- inhibitor-1, which, in the phosphorylated form, is able to inhibits PP1 activity.
Therefore, the binding of the hormone to its receptor triggers a cascade reaction that, among other things, leads to the inhibition of PP1 activity. This maintains phosphorylated both glycogen phosphorylase and glycogen synthase: the first enzyme is activated whereas the latter is inhibited. In this way, the metabolism of carbohydrates is optimized.
Allosteric regulation in muscle and liver
Glycogenolysis is also regulated by both positive and negative allosteric effectors. They act on three enzymes: muscle phosphorylase kinase, hepatic and muscle glycogen phosphorylase, and PP1.
Muscle phosphorylase kinase
Enzyme activity is regulated by two positive allosteric effectors, calcium ion and AMP, and one negative allosteric effector, ATP.
A rise in intracellular calcium ion concentration is the signal for muscle contraction and, once released from the sarcoplasmic reticulum, calcium binds to the delta subunit (calmodulin) of the enzyme, activating it.
AMP accumulates in the muscle during intense contraction, due to the consumption of ATP, and binds to and activates the enzyme. Conversely, when the ATP concentration is high, that is, the muscle is not contracting, it binds to the allosteric site for AMP inactivating the kinase.
Hepatic phosphorylase kinase
Some hormones can act both by triggering covalent modifications of target proteins and by causing the release of calcium ions from the endoplasmic reticulum.
In the liver, phosphorylase kinase is regulated by hormones that cause release of calcium ions. Examples are vasopressin, but also adrenaline when it binds to the alpha1 receptors. With regard to adrenaline, its binding to the alpha1 receptors activates a G protein that stimulates phospholipase C-beta, which in turn converts phosphatidylinositol-4,5-bisphosphate to inositol 1,4,5-triphosphate (IP3) and diacylglycerol. Inositol 1,4,5-triphosphate causes the release of calcium ions from the endoplasmic reticulum. Calcium ions, binding to the delta subunit of phosphorylase kinase, lead to its activation.
Muscle glycogen phosphorylase
Muscle glycogen phosphorylase b is activated in the presence of high concentrations of AMP, which, binding to a specific nucleotide binding site, changes the quaternary structure of the enzyme, shifting the allosteric equilibrium toward the active R state of the b form. Conversely, ATP and glucose-6-phosphate, which compete with AMP for the same nucleotide-binding site, act as negative allosteric effectors, shifting the allosteric equilibrium toward the inactive T state of the b form.
Muscle glycogen phosphorylase a is active, regardless of AMP, ATP, and glucose-6-phosphate levels.
In resting muscle, nearly all the glycogen phosphorylase is in the inactive b form.
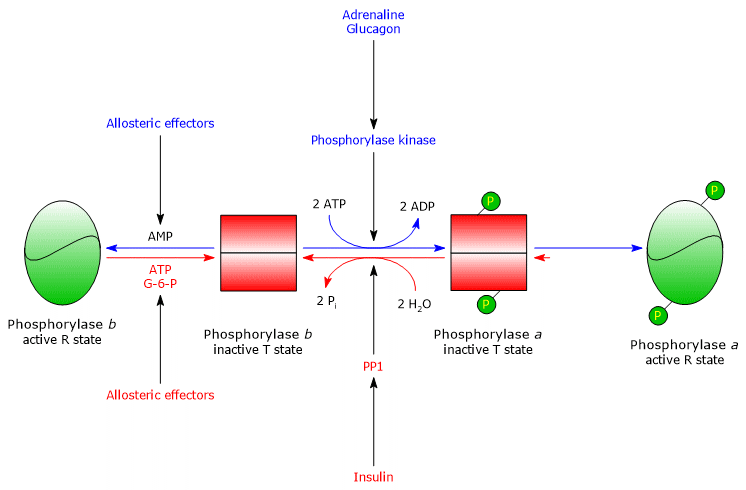
In fact, the covalent and allosteric regulation of the enzyme ensures that intracellular glucose levels are finely regulated.
- If a cell with an adequate energy charge receives the hormonal signal that triggers the cascade of activations, glycogen phosphorylase b, inhibited by ATP and glucose-6-phosphate, remains in the T state until the charge is high.
- If the cellular energy charge is low, glycogen phosphorylase b, activated by AMP, begins glycogen breakdown, even in the absence of the hormonal stimulus that induces its conversion to the active a form.
Allosteric regulation of PP1
PP1 is allosterically activated by glucose-6-phosphate, therefore when the cellular energy charge is low.
Hepatic glycogen phosphorylase
In the liver, the allosteric regulation of the enzyme occurs through different mechanisms.
- The first difference concerns the response to AMP: hepatic glycogen phosphorylase b is not activated by AMP.
- The second difference concerns glycogen phosphorylase a. The enzyme is inhibited by glucose, which is a competitive inhibitor: the monosaccharide shifts the allosteric equilibrium toward the inactive T state of the a form.
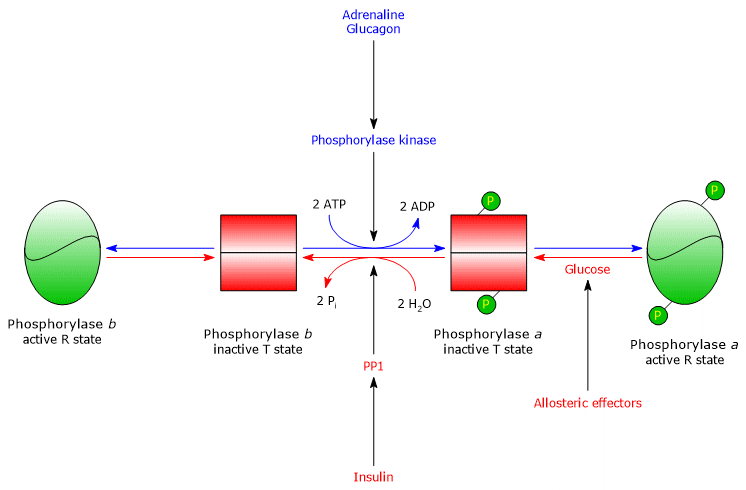
In the liver, the purpose of glycogenolysis is to provide glucose to other tissues when the blood glucose level is low. When blood glucose level is back to normal, its concentration in hepatocytes increases and it binds to glycogen phosphorylase a, inducing a conformational change that exposes the phosphorylated serine residues to PP1 activity, which inactivates the enzyme through dephosphorylation. Therefore, the allosteric site for glucose of hepatic glycogen phosphorylase allows the enzyme to act as a blood glucose sensor, responding appropriately to changes in blood glucose level. Ultimately, the hepatic isoenzyme responds only to glucose, not to AMP, that is, to the cellular energy charge, and this is noteworthy because fatty acids, and not glucose, are the primary energy source for the liver.
References
- Nelson D.L., Cox M.M. Lehninger. Principles of biochemistry. 6th Edition. W.H. Freeman and Company, 2012
- Roach P.J., Depaoli-Roach A.A., Hurley T.D., Tagliabracci V.S. Glycogen and its metabolism: some new developments and old themes. Biochem J 2012:441;763-787. doi:10.1042/BJ20111416
- Rosenthal M.D., Glew R.H. Medical Biochemistry – Human Metabolism in Health and Disease. John Wiley J. & Sons, Inc., Publication, 2009