Docosahexaenoic acid or DHA or cervonic acid or 22:6n-3 is a polyunsaturated fatty acid consisting of a chain of 22 carbon atoms with 6 cis double bonds at positions 4,7,10,13,16, and 19.
Since the first double bond, with respect to the methyl end, is located at carbon 3, the molecule belongs to the group of omega-3 polyunsaturated fatty acids or omega-3 fatty acids, a subclass of lipids.
Like arachidonic acid or ARA, an omega-6 polyunsaturated fatty acid or omega-6 fatty acid, and eicosapentaenoic acid or EPA, another omega-3 fatty acids, docosahexaenoic acid is a long-chain polyunsaturated fatty acid or LC-PUFA.
Among unsaturated fatty acids present in substantial amount in human tissues, it is the most unsaturated and longest one.
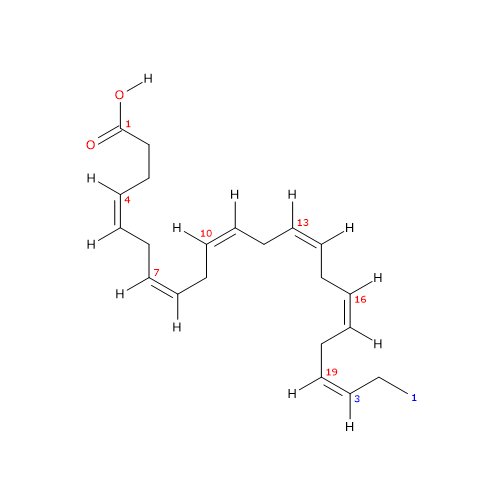
DHA, like EPA and ARA, is found mainly in cell membranes, esterified in phospholipids; hence, like the other two LC-PUFAs, it is a structural components of cellular membranes. It is particularly abundant in neural cells, and is most abundant polyunsaturated fatty acid in the brain.
Many animals, including humans, can synthesize DHA, although with variable efficiency, from alpha-linolenic acid or ALA or 18:3n-3, the precursor to omega-3 polyunsaturated fatty acids and, with linoleic acid or LA or 18:2n-6, one of the two essential fatty acids for animals. In the absence of dietary alpha-linolenic acid, the omega-3 polyunsaturated fatty acids, including DHA, become essential, too, and, for this reason, are defined as conditionally essential fatty acids.
The synthesis of docosahexaenoic acid from alpha-linolenic acid proceeds via elongation and desaturation reactions, and takes place between endoplasmic reticulum and peroxisomes, where the last step occurs, namely, a limited beta-oxidation. The desaturases and elongases that catalyze the conversion of ALA to DHA are shared with the pathways leading to the synthesis of omega-6, omega-7 and omega-9 polyunsaturated fatty acids. There is therefore competition for these enzymes and, at the enzymatic level, omega-3 fatty acids seem to be the preferred substrates for desaturases. However, due to the high linoleic acid content of the Western diet, the synthesis of omega-6 fatty acids seems to be prevalent.
The idea that DHA could be beneficial for human health originated from the work of Hans Olaf Bang and Jorn Dyerberg, who in the early 70s of the last century investigated the reason why thrombotic diseases, particularly ischemic heart disease, were so rare among Greenland Eskimos. These authors suggested that this was due to the their fish-based diet, rich in DHA and EPA, both found in good amounts in the blood of these subjects.
These initial observations led to epidemiological studies, cell culture researches, as well as animal and human feeding studies, searching for the link between DHA and EPA levels and human health.
Adequate dietary intake of DHA and EPA is required throughout development, starting with fetal life, and has been associated with improved health as well as reduced risk of developing diseases, for example cardiovascular diseases, throughout adulthood.
In recent years, the molecular mechanisms underlying the functional effects of DHA are being clarified, that involve:
- an action in non-esterified form on membrane receptors coupled to G proteins;
- an action, in esterified form in the membrane phospholipids, on the fluidity of the membranes, and on the composition of the lipid rafts;
- a role as substrate for the synthesis of different bioactive lipid mediators, such as resolvins, maresins and protectins. These lipid mediators are involved in the regulation of inflammation, platelet aggregation, immunity, smooth muscle contraction and renal function.
For humans, the main dietary sources are fishery products, in particular those from cold waters. However, phytoplankton and not animals is the main producers of docosahexaenoic acid, and eicosapentaenoic acid, in the biosphere: DHA and EPA, going up the food chain, reach our tables.
Fish, molluscs and crustaceans that live in cold waters exploit the anti-freezing effect of EPA and DHA which, due to the degree of unsaturation of the carbon chain, help to regulate the fluidity of cell membranes and act as natural antifreeze agents.
PROPERTIES, NAMES, AND IDENTIFIERS
Molecular weight: 328.496 g/mol
Molecular formula: C22H32O2
Melting point at -44 °C (-47 °F; 229 K)
Boiling point at 446.7 (836.1 °F; 719.8 K)
IUPAC name: (4Z,7Z,10Z,13Z,16Z,19Z)-docosa-4,7,10,13,16,19-hexaenoic acid
CAS registry number: 6217-54-5
PubChem: 445580
European Community (EC) Number: 612-950-9
Contents
- Synthesis
- Where the synthesis take place?
- Cellular distribution
- Mechanisms of action: SPMs
- References
Synthesis
DHA, like arachidonic acid, can only be synthesized from dietary precursors. Below, the metabolic pathway for its synthesis from alpha-linolenic acid through a series of seven steps is analyzed. In this metabolic pathway, we shall meet the other possible precursors.
The first six steps, a series of elongation and desaturation reactions, occur in the endoplasmic reticulum whereas the last one, a beta-oxidation, in peroxisomes.
- In the first step, ALA is desaturated to stearidonic acid or 18:4n-3, in a reaction catalyzed by delta-6 desaturase, also referred to as acyl-CoA 6-desaturase (EC 1.14.19.3). The enzyme requires zinc, magnesium and pyridoxine as cofactors.
- In the second step, stearidonic acid is elongated to 20:4n-3 in a reaction catalyzed by elongase 5 (EC 2.3.1.199, as well as the other Elongases from 1 to 7).
- 20:4n-3 is desaturated to EPA in a reaction catalyzed by delta-5 desaturase (E.C. 1.14.19.44).
- EPA is elongated to docosapentaenoic acid or DPA or 22:5n-3 in a reaction catalyzed by elongase 5 and/or elongase 2 (it is still unclear if one or both elongases catalyze this step).
- DPA is elongated to 24:5n-3 in a reaction catalyzed by elongase 2.
- 24:5n-3 is desaturated to 24:6n-3 in a reaction catalyzed by delta-6 desaturase.
- 24:5n-3 leaves the endoplasmic reticulum and is transferred to peroxisomes where it is converted to docosahexaenoic acid through removal of a two carbon fragment by partial beta-oxidation.
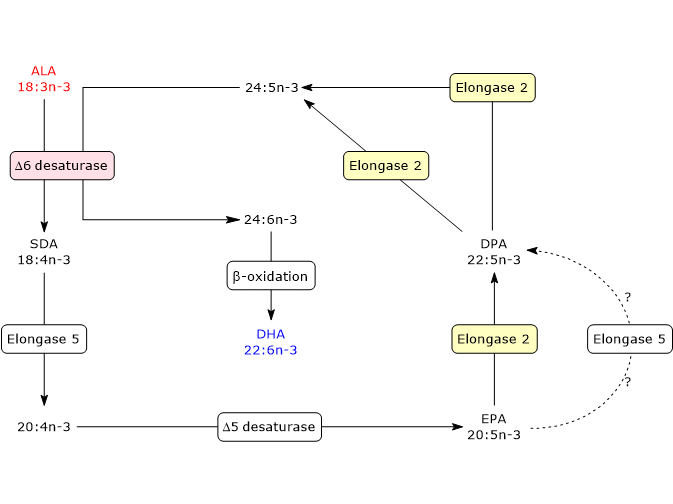
DHA leaves peroxisomes and returns to the endoplasmic reticulum where it is incorporated into membrane phospholipids.
In humans, conversion of alpha-linolenic acid to DHA seems to be very low, ranging from 0 percent to 4 percent. However, higher values, up to 9 percent, have been found in young women, perhaps related to the effects of estrogen on delta-6 desaturase.
A delta-4 desaturase, that allows the synthesis of docosahexaenoic acid directly from 22:5n-3, was identified in some marine microalgae, such as those belonging to the genera Pavlova and Isochrysis, in a lower eukaryote, the marine microheterotroph Thraustochyrium sp., and in a marine, herbivorous, teleost fish, Siganus canaliculatus.
Regulation of the synthesis
The synthesis of docosahexaenoic acid is influenced by factors that act on the catalytic activity of some of the enzymes involved, particularly delta-6 desaturase and elongase 2. Furthermore, since the enzymes catalyzing the synthesis of DHA from ALA are shared with the pathways leading to the synthesis of omega-6, omega-7 and omega-9 fatty acids, these different fatty acids compete for the same enzymes.
Delta-6 desaturase
The enzyme catalyzes both the oxidation of alpha-linolenic acid to stearidonic acid and of 24:5n-3 to 24:6n-3, the most direct precursor of DHA. The first reaction is the rate-limiting step of the pathway.
Delta-6 desaturase, like delta-5 desaturase, is inhibited by cholesterol, saturated fatty acids and trans fatty acids, alcohol, glucocorticoids, and adrenaline. Protein deficiency and total fasting reduce the activity of delta-6 desaturase, whereas its activity is enhanced by partial caloric restriction and insulin.
The competition between alpha-linolenic acid and 24:5n-3 for the desaturase could explain the decrease in DHA levels observed as a result of alpha-linolenic acid supplementation beyond normal intakes.
The activity of both the desaturases is also reduced in diabetes mellitus, hyperlipidemia, hypertension, and metabolic syndrome.
It should be pointed out that, in humans, the activities of delta-5 and delta-6 desaturases are low, with delta-5 desaturase > delta-6 desaturase. Therefore, the conversion of alpha-linolenic acid and linoleic acid to their respective metabolites may be inadequate to the needs under certain conditions, such as in ageing subjects and some diseases.
Elongase 2
The second elongation reaction catalyzed by elongase 2, from DPA to 24:5n-3, seems to be saturated when alpha-linolenic acid, stearidonic acid or EPA are supplied in the diet, suggesting that elongase 2 might play a crucial role in understanding if the synthesis of DHA may be increased by dietary means.
Competition between omega-3, omega-6 and omega-9
Alpha-linolenic acid, linoleic acid and oleic acid are metabolized by the same set of desaturases and elongases. Therefore, there is competition for such enzymes. However, it seems that omega-3 fatty acids are the substrates preferentially metabolized compared to omega-6 fatty acids, and omega-6 fatty acids compared to omega-9 fatty acids, hence with an order of preference: omega-3 > omega-6 > omega-9.
It should be pointed out, however, that linoleic acid is much more abundant than alpha-linolenic acid in the Western diet, as well as in human tissues, and this seems to favor the pathway leading to synthesis of omega-6 polyunsaturated fatty acids compared to the others, although the synthesis of arachidonic acid from linoleic acid is not extremely efficient, too.
Under physiological conditions, omega-9 polyunsaturated fatty acids are present in trivial amounts. This is of great importance as high levels of Mead acid or 20:3n-9 in the plasma point out a deficiency of essential fatty acids, and then of omega-3 and omega-6 fatty acids.
Note that both the omega-3 and omega-6 families inhibit the synthesis of omega-9 polyunsaturated fatty acids.
Where does the synthesis take place?
The main site for the synthesis of DHA is the liver.
The synthesized molecule, after secretion into the bloodstream, becomes available for uptake by other organs, such as the brain.
Among neural cells, namely neurons, astrocytes, oligodendrocytes, and microglia, only astrocytes can synthesize DHA, whereas neurons, which are the major site for its accumulation, can’t synthesized it, lacking desaturase activity.
In astrocytes, the availability of the preformed docosahexaenoic acid negatively influences the synthesis of the fatty acid. Hence, their biosynthetic activity is a minor source for DHA accretion in the brain when the supply of the molecule is adequate.
Finally, it should be noted that brain cerebral-microvascular endothelial cells, although capable to elongate and desaturate fatty acids up to 18 carbons in length, lack the ability to catalyze the final desaturation step in the synthesis of both DHA and 22:5n-6.
Cellular distribution
In most membranes, docosahexaenoic acid is preferentially accumulated in the two major structural phospholipids, namely, phosphatidylethanolamine, and, in lesser amount, phosphatidylcholine. However, this rule does not apply to all cells. For example, in the membranes of neurons, high DHA contents are found in phosphatidylserine, a non-structural phospholipid.
Within membrane phospholipids, DHA is located, like the other PUFAs, mainly in the sn-2 position, whereas sn-1 position is occupied mainly by saturated fatty acids, in particular stearic acid and palmitic acid.
DHA is not homogeneously distributed in tissues and cells, and, based on the amounts present, it is possible to identify two tissue classes: high-DHA tissues and low-DHA tissues.
- Some cells and tissues contain high amounts of docosahexaenoic acid, often up to almost 50 percent of the moles of all acyl chains. Examples are the rod outer segment of the retina, neurons, and sperms. These cells contain so much DHA that, in their membranes, phospholipids with the fatty acid both in sn-1 and sn-2 position are common. And it should be pointed out that, in these cells, DHA levels are not affected by diet.
DHA is the most abundant PUFA in the brain, and numerous studies point out that such levels are essential for optimal function of neurons and retina. Docosahexaenoic acid accretion in the central nervous system occurs during the developmental stage, mainly due to the molecule synthesized in the liver or derived from diet. However, as previously seen, DHA can also be synthesized in the astrocytes. - Other tissues and cells normally contain low amounts of DHA, less than a few mol percent of all acyl chains. In these sites docosahexaenoic acid is present primarily in the sn-2 position of the phospholipids, since it is not present in sufficient amounts to promote esterification in sn-1 position.
In these cells and tissues, DHA levels can be affected by diet. Therefore, it is likely that the health benefits associated with intake of the molecule originate from these tissues.
Mechanisms of action: SPMs
Docosahexaenoic acid has been involved in virtually every human disease. Health benefits have been attributed to cancer treatment, heart disease, neuronal functions, immune problems, aging, but also to other conditions such as sperm fertility, malaria and migraine. Therefore, apparently unrelated abnormalities. This suggests that the action of the molecule occurs at a very basic level, common to many cell types.
DHA may provide health benefits acting in two ways: directly or through its metabolites, of which, currently, over 70 have been described. Indeed, a first molecular explanation was provided for the many health benefits attributed to this fatty acid, as well as to EPA and DPA, different from its direct action. Namely, a new class of lipid mediators, termed specialized pro-resolving mediators or SPMs, has been described. Such molecules have been involved in the active resolution of inflammation, reduction of pain, host defense, and protection of the organ from damage caused by ischemia-reperfusion.
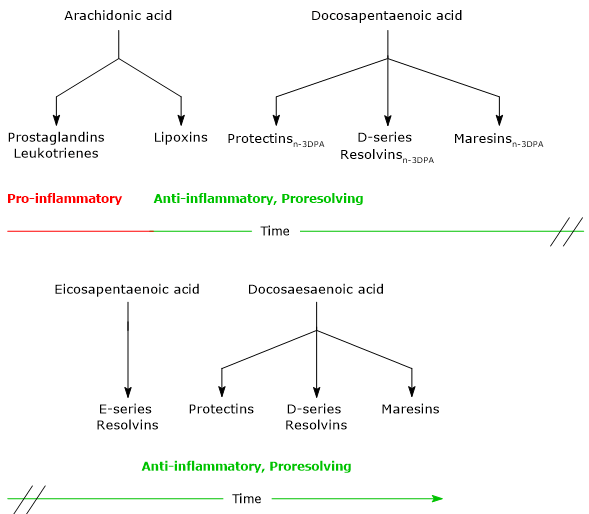
SPMs are synthesized at the site of inflammation or tissue damage through coordinated enzyme-catalyzed reactions, produced in small amounts, some unique to a specific cell or tissue type, such as Maresins in macrophages, and others, whose synthesis involves multiple cell types, to multiple cell or tissue types.
SPMs are able to strongly regulate the activity of target cells. For example, in the course of inflammation, after binding to specific cellular receptors, they regulate cytokine and chemokine production, neutrophil infiltration, and the clearance of apoptotic neutrophils by macrophages, thus promoting the return to tissue homeostasis.
SPMs comprise four families of lipid mediators, below summarized.
- Lipoxins, the first SPMs to be discovered, are synthesized from arachidonic acid.
- Maresins, Protectins and Resolvins, synthesized from EPA, DPA and docosahexaenoic acid.
Noteworthy, ARA is the precursor of both pro-inflammatory and proresolving lipid mediators, whereas EPA, DPA and DHA are precursors of cytoprotective, anti-inflammatory and proresolving lipid mediators.
The synthesis of these mediators follows a precise timeline. Indeed, in acute inflammation, it is possible to identify an initial pro-inflammatory phase, characterized by the synthesis of Leukotrienes and Prostaglandins from arachidonic acid, and a resolution phase, in which Lipoxins, Maresins, Protectins and Resolvins are synthesized.
Docosahexaenoic acid can also undergo a free radical-mediated peroxidation leading to the formation of metabolites, such as Neuroprostanes and Neuroketals, that are produced and act virtually anywhere and at various concentrations.
Secretory phospholipases A2
Docosahexaenoic acid in the site of injury can derive from the circulating blood stream or be released from membrane phospholipids of the involved cells.
- In whole blood of healthy subjects, the amount of DHA is modest, ranging from 1.3 to 5 percent of the total fatty acids, variations attributed to differences in dietary intake. The plasma molecule reaches, within a few minutes from initial damage, the site of the lesion, leaves the circulating blood stream and is available for enzymatic conversion to SPMs, which in turn interact with local immune cells.
- The release of DHA from membrane phospholipids is due to the hydrolytic activity of secretory phospholipases A2 or sPLA2, the first enzymes acting in docosahexaenoic acid metabolism.
Secretory phospholipases A2 are a subfamily of Phospholipases A2 (EC 3.1.1.4), a family of hydrolases comprising, in mammals, more than 30 enzymes, and whose activation occurs in response to specific cell stimuli. sPLA2 account for about one third of all phospholipases A2 and catalyze the release of PUFAs from membrane phospholipids.
LOX, COX-2, sEH, CYP, GST, GGT and dipeptidases
Below is a brief description of other important enzymes involved in DHA metabolism. These enzymes, operating a strict stereochemical control, catalyze the synthesis of molecules with chirality. Note that the configuration of each chiral centers is assigned by the RS system or Cahn-Ingold-Prelog convention.
- Lipoxygenases
They catalyze the synthesis of DHA hydroperoxides by oxygen insertion in the S configuration. These enzymes are 5-lipoxygenase or 5-LOX (EC 1.13.11.34), 12-lipoxygenase or 12-LOX (EC 1.13.11.31), and the 15-lipoxygenase or 15-LOX (EC 1.13.11.33).
5-Lipoxygenase and 12-lipoxygenase also have an epoxide generating activity. - Cyclooxygenase 2
Cyclooxygenase 2 or COX-2 or prostaglandin-endoperoxide synthase 2, together with COX-1, is one of the two isoforms of cyclooxygenases, enzymes able to catalyze the formation of endoperoxides (EC 1.14.99.1 for COX-1 and COX-2).
COX-1 and COX-2 activity is affected by aspirin, which causes an irreversible acetylation of an active-site serine residue. Acetylation of COX-1 leads to loss of activity, thus inhibiting the synthesis of Thromboxanes and Prostaglandins.
Conversely, acetylation of COX-2 does not lead to loss of activity. The enzyme loses cyclooxygenase activity but acquires an enzymatic activity similar to that of 15-lipoxygenase. Indeed, the acetylated enzyme catalyzes, like cytochrome P450, oxygen insertion at position 17, as is the case with 15-LOX, but with opposite stereochemistry, R- rather than S configuration. Therefore, the product of the reaction is 17R-hydroperoxide-DHA rather than 17S-hydroperoxide-DHA. - Soluble epoxide hydrolase
This enzyme (EC 3.3.2.10), abbreviated as sEH, in mammals, is able to catalyze hydrolysis of a broad spectrum of epoxides. - Cytochrome P450
Several members of the cytochrome P450 superfamily are involved in the metabolism of docosahexaenoic acid. The isoforms of cytochrome P450 families 1 to 3 are mainly epoxygenases, and act also on EPA and ARA, whereas the isoforms of the family 4 are mainly omega-hydroxylases. - Other enzymes involved are some members of glutathione S-transferase family or GSTs (EC 2.5.1.18), gamma-glutamyl transferase or GGT (EC 2.3.2.2), and dipeptidases.
Maresins
Maresins or macrophage mediators in resolving inflammation, are a family of compounds with analgesic activity, involved in the resolution of inflammation, and tissue regeneration.
They are produced mainly by macrophages, through biosynthetic pathways that share the first step: oxygenation of DHA at position 14, in a reaction catalyzed by 12-lipoxygenase. The product of this reaction is the hydroperoxy intermediate 14S-hydroperoxy-DHA or 14S-HpDHA or 14S-hydroperoxy-4Z,7Z,10Z,12E,16Z,19Z-docosahexaenoic acid.
Human macrophage 12-lipoxygenase also converts arachidonic acid to the corresponding 14S-hydroperoxide (98 percent), with an efficiency equivalent to that of DHA conversion to 14S-hydroperoxy-DHA.
Hereafter, some examples of Maresins will be discussed.
Maresin 1 or MaR1 or 7R,14S-diHDHA has the E,E,Z conjugated triene motif.
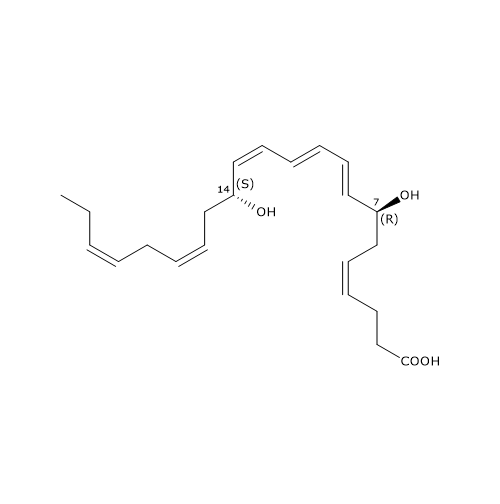
MaR1 was the first member of this family to be identified, and is the product of a pathway that consists of three steps, the first of which has been analyzed in the previous paragraph.
In the second step, 12-lipoxygenase catalyzes the epoxidation of 14S-hydroperoxy-DHA to 13S,14S-epoxy-maresin or 13S,14S-eMaR. This molecule is also a central intermediate in the biosynthesis of Maresin 2 and MCTR1, MCTR3, MCTR3. 13S,14S-eMaR is converted to Maresin 1 in a hydrolase catalyzed reaction leading to the introduction of a hydroxyl group at position 7 and a rearrangement of the double bonds. Maresin 1 is also present in primitive invertebrates, such as Platyhelminthes or flatworms; this suggests that its structure and function have been preserved during evolution.
14S-hydroperoxy-DHA can undergo a second oxygenation catalyzed by 5-lipoxygenase to form 7S,14S-dihydroxy-DHA (E,Z,E), an isomer of Maresin 1.
Maresin 2 or MaR2 or 13R,14S-diHDHA is synthesized via the 13S,14S-epoxy-maresin intermediate, in a reaction catalyzed by sEH. Like Maresin-1, it has a conjugated triene motif, but with Z,E,E geometry.
Maresin conjugates in tissue regeneration
13S,14S-Epoxy-maresin can also be converted to Maresin conjugates in tissue regeneration or MCTRs.
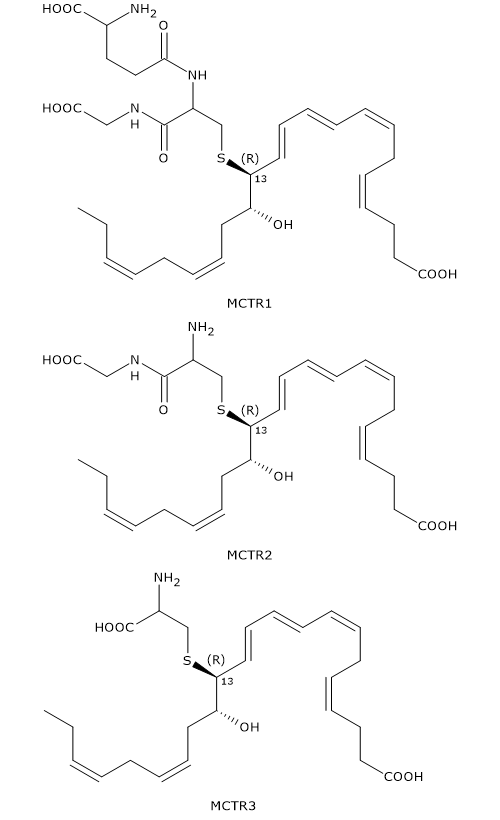
In the first step, 13S,14S-epoxy-maresin reacts with glutathione to form MCTR1 or 13R-glutathion, 14S-HDHA in a reaction that can be catalyzed by two enzymes from the glutathione S-transferase family: leukotriene C4 synthase or LTC4S (EC 4.4.1.20) and GSTM4 (EC 2.5.1.18).
In the second step, gamma-glutamyl transferase catalyzes the cleavage of gamma-glutamyl group from MCTR1 to form MCTR2 or 13R-cysteinylglycinyl,14S-HDHA.
Eventually, in a reaction catalyzed by dipeptidases, the cysteinyl-glycine bond of MCTR2 is cleaved to form the third member of the family: MCTR3 or 13R-cysteinyl,14S-HDHA.
Like Maresin-2, MCTRs have a Z,E,E conjugated triene motif.
Maresin-like lipid mediators
Unlike macrophages, platelets and leukocytes produce docosahexaenoic acid derivatives defined as Maresin-like lipid mediators, in particular Maresin-L1 or 14S-22-diHDHA, and Maresin-L2 or 14R,22-diHDHa.
Below, the synthetic pathways for their production are described.
- Maresin-L1
14S-Hydroperoxy-DHA is reduced to 14S-HDHA, in a reaction catalyzed by a peroxidase.
In the next step, 14S-HDHA is oxidized at position 22 to form Maresin-L1, in a reaction catalyzed by enzymes belonging to the cytochrome P450 family. - Maresin-L2
Cytochrome P450 activity can also convert docosahexaenoic acid to 14R-HDHA.
14R-HDHA can be hydroxylated at position 22 to form Maresin-L2, again, in a reaction catalyzed by cytochrome P450.
14S-HDHA and 14R-HDHA can undergo an alternative n-1 oxidation by cytochrome P450 to form 14S,21S-diHDHA, 14S,21R-diHDHA, 14R,21S-diHDHA, and 14R,21R-diHDHA, respectively.
Cytochrome P450 activity on 14S-HDHA also leads to the formation of 14S,20R-diHDHA.
Protectins
Protectins were discovered during studies on the metabolism of docosahexaenoic acid in brain tissue in response to aspirin treatment, and initially were termed Neuroprotectins. However, as their production and their action are not restricted to neural tissue, the term Protectins was preferred.
They are compounds that, like DHA, have six double bonds. And, like Leukotrienes and several Maresins, three of these double bonds are conjugated, respectively, those at positions 11, 13 and 15, that is, there is a conjugated triene motif. Another distinctive feature is the hydroxyl group at position 17, like Resolvins.
Their molecular structures are highly conserved from fish to humans.
Hereafter, some examples of Protectins.
The most known member of this family is Protectin D1 or PD1 or 10R,17S-diHDHA.
It is the end product of a biosynthesis pathway consisting of three steps.
In the first step, the oxygenation of DHA at position 17 occurs, to form 17S-hydroperoxy-DHA or 17S-HpDHA. The reaction is catalyzed by 15-lipoxygenase and is shared with D-series Resolvin biosynthesis.
In the second step, 17S-HpDHA is epoxidated enzymatically to 16S,17S-epoxy-DHA.
In the last step, the hydrolysis of 16S,17S-epoxy-DHA leads to PD1 formation. The reaction is catalyzed by sEH, with the formation of the correct double bond geometry, namely, E,E,Z.
Protectin conjugates in tissue regeneration
Like Maresins and D-series Resolvins, Protectins can react with glutathione to form a family of compounds called Protectin conjugates in tissue regeneration or PCTRs.
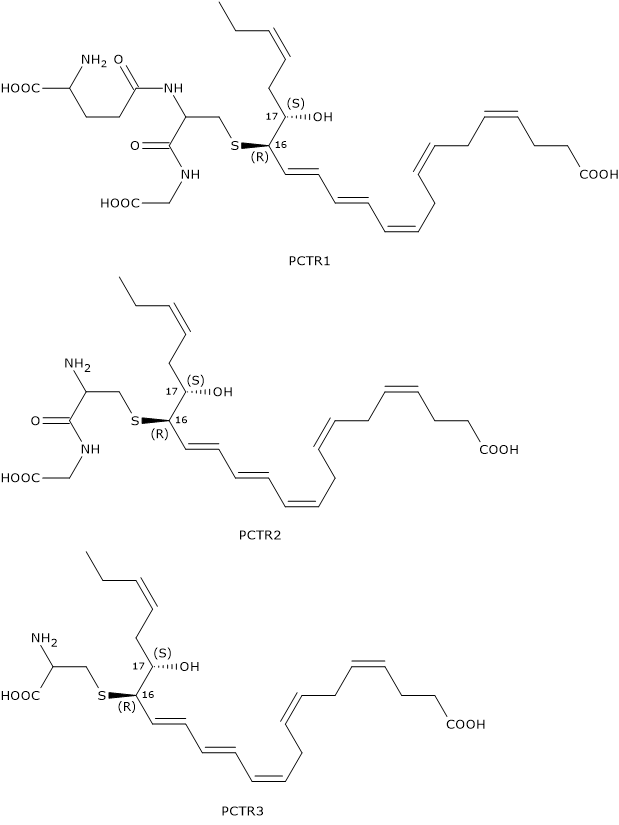
In the first step of PCTR biosynthesis pathway, 16S,17S-epoxy-DHA reacts with glutathione to form PCTR1 in a reaction catalyzed by GST.
In the second step, gamma-glutamyl transferase catalyzes the cleavage of the gamma-glutamyl group from PCTR1 to form PCTR2 or 16R-cysteinylglycinyl,17S-HDHA.
Eventually, in a reaction catalyzed by dipeptidases, the cysteinyl-glycine bond of PCTR2 is cleaved to form the last member of the family: PCTR3 or 16R-cysteinyl,17S-HDHA.
Protectin X
Protectin X or PDX or 10S,17S-dihydroxy-DHA or 10S,17S-diHDHA is an isomer of Protectin D1. The two molecules differ in the:
- configuration of carbon 10: R in PD1 and S in PDX;
- geometry of the conjugated triene motif: E,E,Z for PD1 and E,Z,E for PDX.
The synthesis pathway for PDX consists of several steps and has in common with PD1 synthesis the intermediate 17S-hydroperoxy-DHA.
Then, 17S-hydroperoxy-DHA, in a double di-oxygenation reaction, is converted to PDX. This reaction is catalyzed by 5-lipoxygenase.
PDX is less active than PD1.
Furthermore, 16S,17S-epoxy-DHA can undergo non-enzymatic hydrolysis to form a racemic mixture of two minor products, 16R/S,17S-diHDHA and 10R/S,17S-diHDHA.
Aspirin and Protectins
An additional pathway for the synthesis of Protectins occurs in response to aspirin treatment, and to its effect on COX-2 catalytic activity. The acetylated enzyme catalyzes the insertion of oxygen at position 17 of docosahexaenoic acid, but with R– rather than S-configuration. Therefore, DHA is converted to 17R-HpDHA, and not to 17S-HpDHA, and all the derived Protectins, called aspirin-triggered protectins or AT-protectins and synthesized according to the pathways described above, have the R configuration at position 17.
Examples are:
- AT-PD1;
- the racemic mixture of 10R/S,17R-diHDHA and 16R/S,17R-diHDHA;
- 10S,17R-diHDHA.
COX-2 can also catalyze the oxidation of arachidonic acid to form 15R-HETE. In turn, 15R-HETE is converted to Lipoxins, the first lipid mediators to initiate the resolution of inflammation.
D-series Resolvins
D-series Resolvins or RvDs are a family of compounds composed of six molecules designated RvD1 through RvD6. They exhibit many beneficial effects such as anti-inflammatory, neuroprotective and proresolving activities
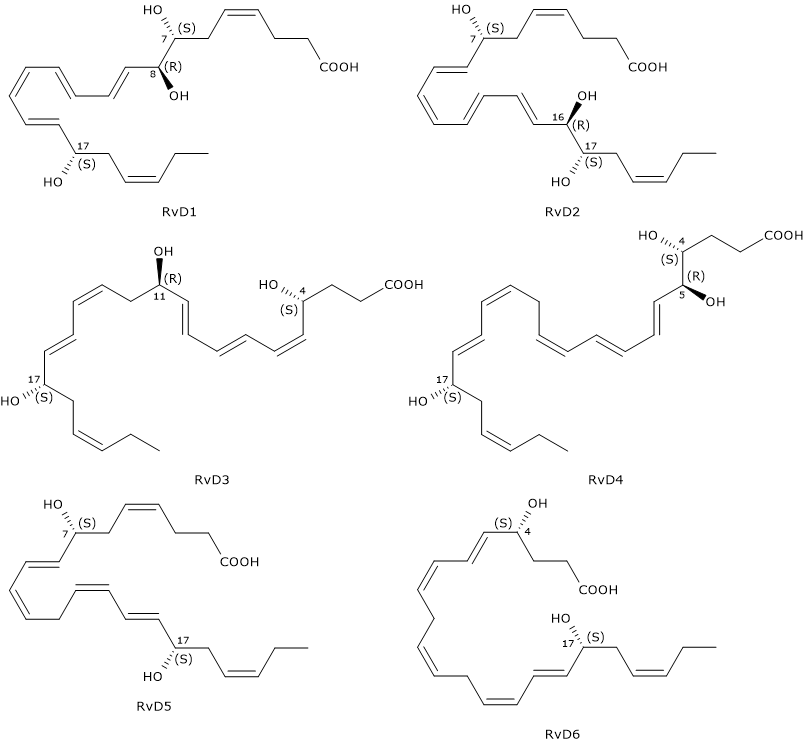
D-series Resolvins have six double bonds of which, in RvD1 and RvD2, four are conjugated to form a E,E,Z,E and E,Z,E,E conjugated tetraene motif, respectively.
Another distinctive feature is that RvDs are, with the exclusion of RvD5 and RvD6, trihydroxylated compounds.
Furthermore, like Protectins, they have a hydroxyl group at position 17, also in this case, due to the conversion of docosahexaenoic acid to the intermediate 17S-hydroxyperoxide-DHA, in a reaction catalyzed by 15-lipoxygenase, namely, Protectin and Resolvin synthesis pathways share the first step.
Below, the successive steps leading to the synthesis of the six D-series Resolvins.
- 17S-Hydroperoxy-DHA is reduced to 17S-hydroxy-DHA, in a reaction catalyzed by a peroxidase.
- 17S-Hydroxy-DHA is converted to 4S-hydroperoxy, 17S-hydroxy-DHA and 7S-hydroperoxy, 17S-hydroxy-DHA, respectively. The reaction is catalyzed by 5-lipoxygenase.
- In the next step, the two intermediates are converted to 4S,5S-, and 7S,8S-epoxy, 17S-hydroxy-DHA, respectively. Epoxide synthesis is due to the 5-lipoxygenase epoxide generating activity.
- The hydrolysis of the epoxides, catalyzed by a hydrolase, leads to the formation of the D-series Resolvin 1 to 4: RvD1 and the epimer RvD2 from 7S,8S-epoxy, 17S-hydroxy-DHA, and RvD3, and the RvD4 epimer from 4S,5S-epoxy, 17S-hydroxy-DHA.
- The synthesis of RvD5 and RvD6 follows a different route, that is, they are produced from 7S-hydroperoxy, 17S-hydroxy-DHA and 4S-hydroperoxy, 17S-hydroxy-DHA, respectively, in a peroxidase-catalyzed reaction.
Note: also EPA can be converted enzymatically to a family of compounds called E-series Resolvins or RvEs. D and E nomenclatures derives from DHA and EPA, respectively.
Resolvin conjugates in tissue regeneration
Like Maresins and Protectins, D-series Resolvins can react with glutathione to form a family of compounds called Resolvin conjugates in tissue regeneration or RCTRs.
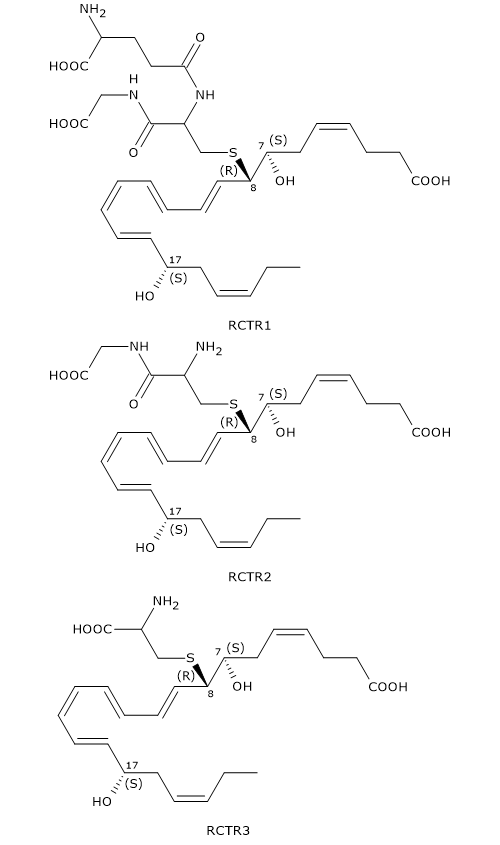
Their synthesis seems to follow the same pattern as for MCTR and PCTR synthesis.
In the first step, 7S,8S-epoxy, 17S-hydroxy-DHA reacts with glutathione to form RCTR1 or 8R-glutathionyl-7S,17S-diHDHA. The reaction is catalyzed by GST.
In the second step, the gamma-glutamyl group is cleaved from RCTR1 to form RCTR2 or 16R-cysteinylglycinyl,7S,17S-diHDHA. The reaction is catalyzed by gamma-glutamyl transferase.
Eventually, in a reaction catalyzed by dipeptidases, the cysteinyl-glycine bond of RCTR2 is cleaved to form the last member of the family: RCTR3 or 8R-cysteinyl,7S,17S-diHDHA.
Aspirin and Resolvins
Aspirin-acetylated COX-2 catalyzes the synthesis of 17R D-series Resolvins or aspirin-triggered (AT) resolvins, designated AT-RvD1 through AT-RvD6.
Such stereoisomers, like Maresin-L2, 14S,20R-diHDHA, 14R,21S-diHDHA and 14R,21R-diHDHA, can derive from cytochrome P450 pathway, too.
Receptors for docosahexaenoic acid and its derivatives
DHA may provide health benefits acting either directly or through its metabolites.
Docosahexaenoic acid is a ligand of PPARalpha, a nuclear receptor, but can also bind to the transmembrane protein G protein-coupled receptor 120 or GPR120, also known as Free fatty acid receptor 4 or FFAR4.
On the other hand, DHA metabolites are molecules that, after binding to specific cell membrane receptors, have a wide range of actions and act simultaneously at different sites and levels.
Here are some examples of receptors for D-Series Resolvins.
- ALX/FRP2, with which RvD1 and AT-RvD1 interact;
- G-protein coupled receptor 18 or GPR18 or RvD2 receptor or DRV1, to which RvD2 binds;
- G-protein coupled receptor 32 or GPR32, also known as RvD1 receptor or DRV1, to which RvD1, its epimers At-RvD1, RvD3 and RvD5 bind.
References
- Burdge G.C, Jones A.E, Wootton S.A. Eicosapentaenoic and docosapentaenoic acids are the principal products of α-linolenic acid metabolism in young men. Br J Nutr 2002;88(4):355-364. doi:10.1079/BJN2002662
- Burdge G.C., Wootton S.A. Conversion of α-linolenic acid to eicosapentaenoic, docosapentaenoic and docosahexaenoic acids in young women. Br J Nutr 2002;88(4):411-420. doi:10.1079/BJN2002689
- Dalli J., Vlasakov I., Riley I.R., Rodriguez A.R., Spur B.W., Petasis N.A, Chiang N., and Serhan C.N. Maresin conjugates in tissue regeneration biosynthesis enzymes in human macrophages. Proc Natl Acad Sci U S A 2016;113(43):12232-12237. doi:10.1073/pnas.1607003113
- Das U.N. Essential fatty acids: biochemistry, physiology and pathology. Biotechnol J 2006;1;420-439. doi:10.1002/biot.200600012
- Deng B., Wang C.W., Arnardottir H.H., Li Y., Cheng C.Y., Dalli J., Serhan C.N. Maresin biosynthesis and identification of maresin 2, a new anti-inflammatory and proresolving mediator from human macrophages. PLoS One 2014;9(7):e1023629. doi:10.1371/journal.pone.0102362
- Duvall M.G., Levy B.D. DHA- and EPA-derived resolvins, protectins, and maresins in airway inflammation. Eur J Pharmacol 2016;785:144-155. doi:10.1016/j.ejphar.2015.11.001
- Dyerberg J., Bang H.O., Hjorne N. Fatty acid composition of the plasma lipids in Greenland Eskimos. Am J Clin Nutr 1975:28;958-966. doi:10.1093/ajcn/28.9.958
- Giltay E.J., Gooren L.J., Toorians A.W., Katan M.B., Zock P.L. Docosahexaenoic acid concentrations are higher in women than in men because of estrogenic effects. Am J Clin Nutr 2004;80(5):1167-1174. doi:10.1093/ajcn/80.5.1167
- Gregory M.K., Gibson R.A., Cook-Johnson R.J., Cleland L.G., James M.J. Elongase reactions as control points in long-chain polyunsaturated fatty acid synthesis. PLoS ONE 2011; 6(12):e29662. doi:10.1371/journal.pone.0029662
- Hong S., Lu Y., Tian H., Alapure B.V., Wang Q., Bunnell B.A., Laborde J.M. Maresin-like lipid mediators are produced by leukocytes and platelets and rescue reparative function of diabetes-impaired macrophages. Chem Biol 2014;21:1318-1329. doi:10.1016/j.chembiol.2014.06.010
- Kim H-Y. Novel metabolism of docosahexaenoic acid in neural cells. J Biol Chem 2007;282(26):18661-18665. doi:10.1074/jbc.R700015200
- Kuda O. Bioactive metabolites of docosahexaenoic acid. Biochimie 2017;136:12-20. doi:10.1016/j.biochi.2017.01.002
- Lee J.M., Lee H., Kang S. and Park W.J. Fatty acid desaturases, polyunsaturated fatty acid regulation, and biotechnological advances. Nutrients 2016;8(1):23. doi:10.3390/nu8010023
- Rodriguez A.R., Spur B.W. Total synthesis of pro-resolving and tissue-regenerative Protectin sulfido-conjugates. Tetrahedron Lett 2015;56(42):5811-5815. doi:10.1016/j.tetlet.2015.09.020
- Serhan C.N., Dalli J., Colas R.A., Winkler J.W., Chiang N. Protectins and maresins: new pro-resolving families of mediators in acute inflammation and resolution bioactive metabolome. Biochim Biophys Acta 2015;1851(4):397-413. doi:10.1016/j.bbalip.2014.08.006
- Serhan C.N. and Petasis N.A. Resolvins and protectins in inflammation resolution. Chem Rev 2011;111:5922-5943. doi:10.1021/cr100396c
- Stillwell W. Docosahexaenoic acid: a most unusual fatty acid. Chem Phys Lipids 2008;153(1):1-2. doi:10.1016/j.chemphyslip.2008.02.012
- Weylandt K.-H. Docosapentaenoic acid derived metabolites and mediators – The new world of lipid mediator medicine in a nutshell. Eur J Pharmacol 2016;785:108-115. doi:10.1016/j.ejphar.2015.11.002