The glucose-alanine cycle, or Cahill cycle, proposed for the first time by Mallette, Exton and Park, and Felig et al. between 1969 and 1970, consists of a series of steps through which extrahepatic tissues, for example the skeletal muscle, export pyruvate and amino groups as alanine to the liver, and receive glucose from the liver via the bloodstream.[1][4]
The main steps of the glucose-alanine cycle are summarized below.[5][6]
- When in extrahepatic tissues amino acids are used for energy, pyruvate, derived from glycolysis, is used as amino group acceptor, forming alanine, a nonessential amino acid.
- Alanine diffuses into the bloodstream and reaches the liver.
- In the liver, the amino group of alanine is transferred to alpha-ketoglutarate to form pyruvate and glutamate, respectively.
- The amino group of glutamate mostly enters the urea cycle, and in part acts as a nitrogen donor in many biosynthetic pathways.
Pyruvate enters gluconeogenesis and is used for glucose synthesis. - The newly formed glucose diffuses into the bloodstream and reaches the peripheral tissues where, due to glycolysis, is converted into pyruvate that can accept amino groups from the free amino acids, thus closing the cycle.
Therefore, the glucose-alanine cycle provides a link between the metabolism of carbohydrates and amino acids, as schematically described below.[6]
Glucose → Pyruvate → Alanine → Pyruvate → Glucose
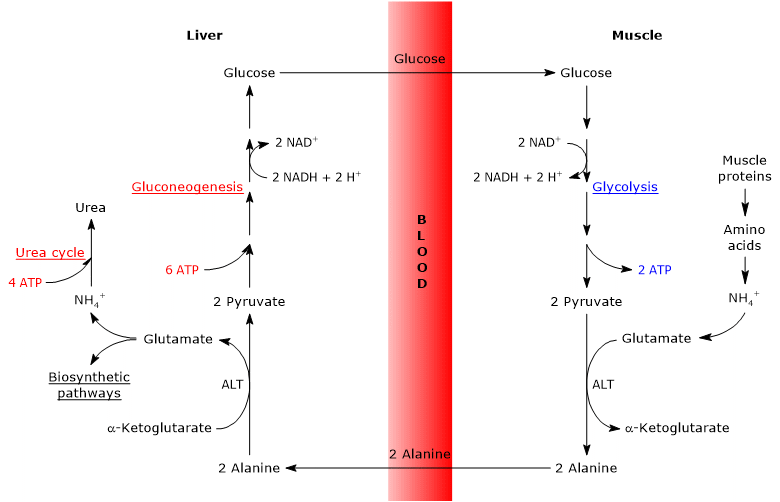
The glucose-alanine cycle occurs not only between the skeletal muscle, the first tissue in which it was observed, and the liver, but involves other cells and extrahepatic tissues including cells of the immune system, such as lymphoid organs.
Contents
Steps
The analysis of the steps of the glucose-alanine cycle is made considering the cycle between skeletal muscle and the liver.
Both intracellular and extracellular proteins are continuously hydrolyzed to the constituent amino acids and resynthesized, and the rate at which these processes occur is balanced precisely, thereby preventing loss of fat free mass.[3]
However, under catabolic conditions, such as intense and prolonged exercise or fasting, the rate of muscle protein breakdown exceeds synthesis. This leads to the liberation of amino acids, some of which are used for energy and others for gluconeogenesis. And the oxidation of the carbon skeletons of amino acids, in particular branched chain amino acids or BCAA, leucine, isoleucine and valine, may be a significant source of energy for the muscle. For example, after about 90 minutes of strenuous exercise, amino acid oxidation in muscle provides 10-15 percent of the energy needed for contraction.
The utilization of the carbon skeletons of amino acids for energy involves the removal of the amino group, and then the excretion of amino nitrogen in a non-toxic form.[5][6]
The removal of the alpha-amino group occurs by transamination, that can be summarized as follows:
alpha-Keto acid + Amino acid ⇄ New amino acid + New alpha-keto acid
Such reactions, catalyzed by enzymes called aminotransferases or transaminases (EC 2.6.1-) are freely reversible.
Branched chain amino acids, for example, transfer the amino group to alpha-ketoglutarate or 2-oxoglutaric acid, to form glutamate and the α-keto acid derived from the original amino acid, in a reaction catalyzed by branched chain aminotransferase or BCAT (EC 2.6.1.42).
The glucose-alanine cycle in skeletal muscle
In skeletal muscle, the newly formed glutamate may react with ammonia to form glutamine, for many tissues and organs, such as the brain, the major vehicle for interorgan transport of nitrogen.[7] The reaction is catalyzed by the cytosolic enzyme glutamine synthetase (EC 6.3.1.2), and consumes an ATP.
Glutamate + NH4+ + ATP → Glutamine + ADP + Pi
In this case, glutamate leaves the Cahill cycle.
Alternatively, and in contrast to what happens in most of the other tissues, the newly formed glutamate may transfer the amino group to pyruvate, derived from glycolysis, to form alanine and alpha-ketoglutarate. This transamination is catalyzed by alanine aminotransferase or ALT (EC 2.6.1.2), an enzyme found in most animal and plant tissues.
Glutamate + Pyruvate ⇄ Alanine + alpha-Ketoglutarate
The alanine produced and that derived directly from protein breakdown, and muscle proteins are rich in alanine, can leave the cell and be carried by the bloodstream to the liver; in this way the amino group reaches the liver. And the rate at which alanine formed by transamination of pyruvate is transferred into the circulation is proportional to the intracellular pyruvate production.
Note: Alanine and glutamine are the major sources of nitrogen and carbon in interorgan amino acid metabolism.
The glucose-alanine cycle in the liver
Once in the liver, a hepatic alanine aminotransferase catalyzes a transamination in which alanine, the major gluconeogenic amino acid, acts as an amino group donor and alpha-ketoglutarate as an alpha-keto acid acceptor.[6] The products of the reaction are pyruvate, i.e. the carbon skeleton of alanine, and glutamate.
Alanine + alpha-Ketoglutarate ⇄ Glutamate + Pyruvate
Glutamate, in the reaction catalyzed by glutamate dehydrogenase (EC 1.4.1.2), an enzyme present in the mitochondrial matrix, forms ammonium ion, which enters the urea cycle, and alpha-ketoglutarate, which can enter the Krebs cycle. This reaction is an anaplerotic reaction that links amino acid metabolism with the citric acid cycle.[6]
Glutamate + H2O + NAD+ ⇄ alpha-Ketoglutarate + NH4+ + NADH + H+
However, glutamate can also react with oxaloacetate to form aspartate and alpha-ketoglutarate, in a reaction catalyzed by aspartate aminotransferase (EC 2.6.1.1). Aspartate is involved in the formation of urea as well as in the synthesis of purines and pyrimidines.
Glutamate + Oxaloacetate ⇄ Aspartate + alpha-Ketoglutarate
Also the pyruvate produced may have different metabolic fates: it can be oxidized for ATP production, and then leave the glucose-alanine cycle, or enter the gluconeogenesis pathway, and thus continue in the cycle.
The glucose produced is released from the liver into the bloodstream and delivered to various tissues that require it, as the skeletal muscle, in which it is used for pyruvate synthesis. In turn, the newly formed pyruvate may react with glutamate, thus closing the cycle.
Transaminases
As previously mentioned, the removal of the amino group from amino acids occurs through transamination. These reactions are catalyzed by enzymes called aminotransferases or transaminases.[6]
They are cytosolic enzymes, present in all cells and particularly abundant in the liver, kidney, intestine and muscle; they require pyridoxal phosphate or PLP, the active form of vitamin B6 or pyridoxine, as a coenzyme, which is tightly bound to the active site.
In transamination reactions, the amino group of free amino acids, except of threonine and lysine, is channeled towards a small number of keto acids, notably pyruvate, oxaloacetate and alpha-ketoglutarate.
Cells contain different types of aminotransferases: many are specific for alpha-ketoglutarate as alpha-keto acid acceptor, but differ in specificity for the amino acid, from which they are named. Examples are the aforementioned alanine aminotransferase, also called alanine transaminase and glutamic pyruvic transferase or GPT, and aspartate aminotransferase or AST, also called glutamic-oxaloacetic transaminase or GOT.
It should be underlined that there is no net deamination in these reactions, no loss of amino groups, as the alpha-keto acid acceptor is aminated and the amino acid deaminated.
Functions
This cycle has various functions.[2][5][7]
- It transports nitrogen in a non-toxic form from peripheral tissues to the liver.
- It transports pyruvate, a gluconeogenic substrate, to the liver.
- It removes pyruvate from peripheral tissues. This leads to a higher production of ATP from glucose in these tissues. In fact, the NADH produced during glycolysis can enter the mitochondria and be oxidized through oxidative phosphorylation.
- It allows to maintain a relatively high concentration of alanine in hepatocytes, sufficient to inhibit protein degradation.
- It may play a role in host defense against infectious diseases.
Finally, it is important to underline that there is no net synthesis of glucose in the glucose-alanine cycle.
Energy cost
Like the Cori cycle, also the glucose-alanine cycle has an energy cost, equal to 3-5 ATP.
The part of the cycle that takes place in peripheral tissues involves the production of 5-7 ATP per molecule of glucose:
- 2 ATP are produced by glycolysis;
- 3-5 ATP derive from NADH/FADH2.
Instead in the liver, gluconeogenesis and the urea cycle cost 10 ATP:
- 6 ATP are consumed in the during gluconeogenesis per molecule of glucose synthesized;
- 4 ATP are consumed in the urea cycle per molecule of urea synthesized.
The glucose-alanine cycle, like the Cori cycle, shifts part of the metabolic burden from extrahepatic tissues to the liver.[6] However, the energy cost paid by the liver is justified by the advantages that the cycle brings to the whole body, as it allows, in particular conditions, an efficient breakdown of proteins in extrahepatic tissues, especially skeletal muscle, which in turn allows to obtain gluconeogenic substrates as well as the use of amino acids for energy in extrahepatic tissues.
Similarities and differences with the Cori cycle
There are some analogies between the two cycles, which are listed below.[6][7]
- The Cahill cycle partially overlaps the Cori cycle when pyruvate is converted to glucose and the monosaccharide is transported to extrahepatic tissues, in which it is converted again to pyruvate via the glycolytic pathway.
- The entry into gluconeogenesis pathway is similar for the two cycles: both alanine and lactate are converted to pyruvate.
- Like the Cori cycle, the glucose-alanine cycle occurs between different cell types, unlike metabolic pathways such as glycolysis, citric acid cycle or gluconeogenesis that occur within individual cells
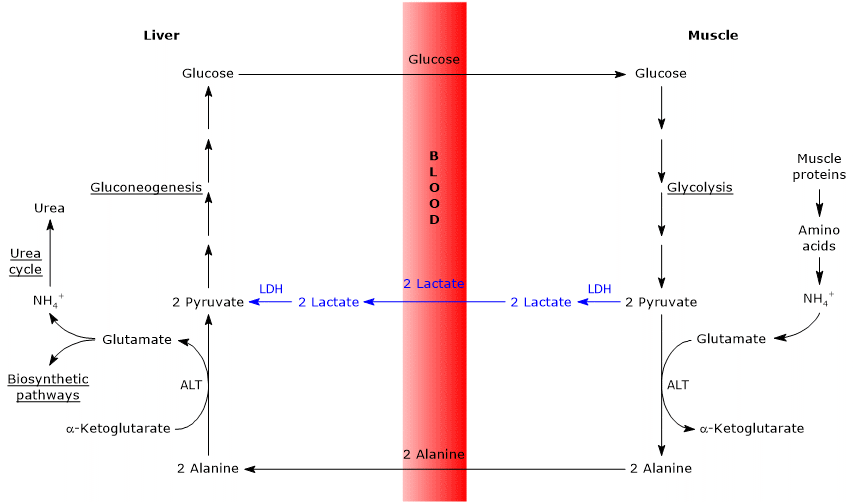
Below, some differences between the two cycles.
- The main difference concerns the three carbon intermediate that from peripheral tissues reach the liver: lactate in the Cori cycle, and alanine in the glucose-alanine cycle.
- Another difference concerns the fate of the NADH produced by glycolysis in peripheral tissues.
In the Cori cycle, the coenzyme acts as reducing agent to reduce pyruvate to lactate, in the reaction catalyzed by lactate dehydrogenase (EC 1.1.1.27).
In the glucose-alanine cycle, this reduction does not occur and the electrons of NADH can be transported into the mitochondria via the malate-aspartate and glycerol 3-phosphate shuttles, generating NADH, the first shuttle, and FADH2, the other shuttle. And the yield of ATP from NADH and FADH2 is 2.5 and 1.5, respectively. - Finally, from the previous point, it is clear that, unlike the Cori cycle, the Cahill cycle requires the presence of oxygen and mitochondria in the peripheral tissues.
References
- ^ Felig P., Pozefsk T., Marlis E., Cahill G.F. Alanine: key role in gluconeogenesis. Science 1970;167(3920):1003-1004. doi:10.1126/science.167.3920.1003
- ^ Gropper S.S., Smith J.L., Groff J.L. Advanced nutrition and human metabolism. Cengage Learning, 2009
- ^ Lecker S.H., Goldberg A.L. and Mitch W.E. Protein degradation by the ubiquitin–proteasome pathway in normal and disease states. J Am Soc Nephrol 2006;17(7):1807-1819.doi:10.1681/ASN.2006010083
- ^ Mallette L. E., Exton J.H., and Park C.R. Control of gluconeogenesis from amino acids in the perfused rat liver. J Biol Chem 1969;244(20):5713-5723. doi:10.1016/S0021-9258(18)63618-X
- ^ a b c Moran L.A., Horton H.R., Scrimgeour K.G., Perry M.D. Principles of Biochemistry. 5th Edition. Pearson, 2012
- ^ a b c d e f g h Nelson D.L., Cox M.M. Lehninger. Principles of biochemistry. 6th Edition. W.H. Freeman and Company, 2012
- ^ a b c Wu G. Amino acids: biochemistry and nutrition. CRC Press, 2010